William LEVENGOOD,
et al
Electroculture
http://www.iccra.org/levengood/allotherpub.htm
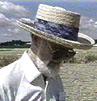
William LEVENGOOD
Journal of Experimental Botany.1975; 26: 911-919
W.C. LEVENGOOD, JUDITH BONDIE and CHI-LING CHEN,
Biophysical Research Department, Sensors, Inc.
3908 Varsity Drive, Ann Arbor, Michigan 48104
Seed Selection for Potential
Viability.
A simple method is presented for selecting individual seeds for
growth and vigour prior to germination. The selection is based on
the measurement of an electric current originating during the
initial stage of seed imbibition. After selecting, seeds may be
returned to the quiescent state without affecting viability.
Results of both laboratory germination and field experiments
demonstrate that high germination rates, more extensive growth,
higher yields, and fewer defective plants are associated with
small currents. Seeds of low viability and high currents may be
removed to upgrade the germination and vigour. Results are
presented from several varieties of Phaseolus vulgaris and from
three other plant species. Experiments are discussed which
indicate that seeds graded by current levels possess markedly
different respiration rates.
Method and apparatus for enhancing
growth characteristics of seeds using ion-electron
avalanches
US6023880
[ PDF ]
Also published as: US5740627 //
WO9811770 // JP2000502911 // JP3213329
Abstract
A method and apparatus for treating seeds with self-organized
avalanches of electrons between electrodes (11, 12) as a cathode
and an anode with seeds (13) between the anode and cathode or on
the anode. Apparatus circuit (200) in a box (20) provides
simultaneous DC and AC between the electrodes which creates the
avalanche of electrons which project into the seeds. The seeds
must be stored before planting. The seeds so treated have enhanced
growth characteristics.
BACKGROUND OF THE INVENTION
(1) Field of the Invention
The present invention relates to a method and apparatus for
treating seeds, thereby reproducibly enhancing rate and uniformity
of seed germination, early growth, root growth, maturity, and
yield in food crops and other plants. These results are achieved
by exposing seeds or growing plants to uniform,
spontaneously-organized pulses of ion-electron avalanches. One
important aspect is allowing a period of several weeks storage
before planting thereby allowing internal, biochemical changes to
take place at the cellular level within the seed. The present
invention also relates to a quality-control method and apparatus
for selecting optimal treatment parameters with the avalanches of
ions and electrons for each variety of seed.
(2) Description of Related Art
Almost since the discovery of the commercial use of electricity,
experimenters have tried to electrically influence plant growth.
Various prior art experimenters have claimed positive results from
exposing growing plants to electrical stimulation in situ. A
wiring network over a field of growing crops is not cost-effective
or practical on a commercial scale, and such techniques have not
been adopted by farmers.
Some prior art experimenters have attempted to avoid the
prohibitive cost of wiring a field by applying electromagnetic
treatments to seeds before planting. Despite reports of increased
growth and, in some cases, increased yield, these results have
proven difficult to repeat and have not achieved commercial use.
Parry (U.S. Pat. No. 2,308,204 (1943)) describes the use
of an oscillating DC voltage to treat seeds to increase
germination of the seeds. There is no indication of improved
plants. Jonas (U.S. Pat. No. 2,712,713 (1955)) and others
exposed seeds to high frequency oscillating fields between 30 MHz
and microwave range, claiming faster and more uniform germination.
Jonas stated that the work of others along similar lines have been
impossible to repeat and confirm. The patent describes only
increased germination of the seeds. Amburn (U.S. Pat. Nos.
3,675,367 (1972) and 3,765,125 (1975)) exposed seeds
to magnetic fields, claiming increased germination rate as an
effect. Because of unreliability and non-reproducibility, none of
these methods have achieved widespread commercial acceptance.
Levengood (U.S. Pat. No. 3,822,505 (1974)) describes an
apparatus for genetically altering plant cells using combined
electrical and magnetic fields. The electrical field is static.
There was alteration in the growth of seeds, but the method was
not repetitively effective from batch to batch of seeds. Another
patent to Levengood (U.S. Pat. No. 3,852,914 (1974))
describes a method for testing seeds for viability, by measuring
pregermination tissue conductivity.
Schiller et al (U.S. Pat. No. 4,633,611 (1987)) describe
treating seeds to disinfect them with low energy electrons using
an electron gun. The radiation dosages are quite high and the
acceleration voltages are between 25 and 75 kV. The use of high
energy ionizing radiation can cause damage to chromosomes and
resultant genetic change which poses complications for use in open
fields. There is no indication that the growth of the plant is
enhanced on a reproducible basis. Yoshida (U.S. Pat. No.
4,758,318 (1988)) describes using a pulsating direct current
to prevent mold. The voltages were 300 to 20,000 V DC which were
pulsed. This method is not practical on a large scale and the
results were variable. Liboff et al (U.S. Pat. No. 5,077,934
(1992)) describe the use of magnetic fields with plants in the
soil. This method is not practical.
Levengood (U.S. Pat. No. 5,288,626 (1994)) describes
genetically transferring DNA between plants using a constant DC
voltage. This is also described in Bioelectrochemistry and
Bioenergetics (1991). These are techniques for producing
genetically altered plants.
Other patents of general interest are Saruwatari (U.S. Pat. No.
4,188,751 (1980)) relating to magnetic treatment; Weinberqer
(U.S. Pat. No. 3,703,051 (1972)) relating to ultrasound; U.S.
Pat. No. 3,940,885 (1976) relating to microwaves.
One system which used an A.C. ripple in a D.C. current to produce
pulses is Tellefson (U.S. Pat. No. 5,117,579 (1992)).
Pulses of ions were produced from wire brush emitters to flood
growing plants in a field. The method is not used with seeds.
There is clearly a need for a reproducible and reliable method for
treating seeds to enhance their growth characteristics. The prior
art methods have not met this need since no such method is used
commercially.
OBJECTS
It is therefore an object of the present invention to provide an
improved, reproducible method and apparatus for enhancing the
growth characteristics of seeds. It is further an object of the
present invention to provide such a method which is simple,
reliable and economical to perform. Further still it is an object
of the present invention to provide a method and apparatus for
detecting whether or not the treated seeds have been effectively
improved in their growth characteristics by the method and
apparatus for enhancing growth characteristics Further still, it
is an object of the present invention to provide a method and
apparatus which allows monitoring during treatment of the
effectiveness of the apparatus for performing the treatment. These
and other objects will become increasingly apparent by reference
to the following specification and the drawings.
BRIEF DESCRIPTION OF DRAWINGS
FIG. 1A is a schematic view of the apparatus of the present
invention for producing controlled, spontaneous, electrostatic
pulses which form the organized electron avalanches between an
anode electrode 11 supporting seeds 13 and a cathode electrode
12.
FIG. 1B is a charted graph showing organized electron
avalanches produced in the apparatus of FIG. 1A with different
DC voltages (relative humidity 26%; p =1009.3 mb).
FIGS. 2A, 2B and 2C are graphs showing growth differences
in tomatoes, pepper and carrot using a DC voltage for five
minutes in the apparatus of FIG. 1A with seeds stored for 35 or
36 days. Germination data was taken at the 12-day growth stage
and represents hypocotyl extension (seedlings placed under grow
lights at 4-day development). The data was compared with two
control sets in each test series. FIG. 2A shows tomato seeds
tested 35 days after exposure. FIG. 2B shows pepper seeds tested
35 days after exposure. FIG. 2C shows carrot seeds tested 36
days after exposure. As can be seen, similar curve shapes appear
in the 5-minute exposure data. In every case the maximum peak is
at the 5-kV level, with a secondary peak at 20-KV.
FIGS. 3A and 3B are graphs showing redox ratio (ratio of
active anions to cations) changes in developing wheat and maize
seedlings over a 60 minute test interval in both untreated,
control seed and in seed exposed to the spontaneously organized
ion-electron avalanches, with avalanche exposure of 30 seconds
at 10 kV (FIG. 3A) and 20 kv (FIG. 3B) The seeds were stored for
eight (8) days. The leaf tissue between electrodes 11 and 12 was
tested after 12 days under a grow light.
FIG. 4 is a graph showing redox ratio changes in mature,
field grown carrot foliage from both untreated control seeds and
seeds exposed to ion-electron avalanches at 5 kV for 5 minutes
and stored for 81 days before planting. Redox Ratio: FIG. 4
shows redox ratios of MIR-treated carrots to be lower than that
of untreated controls, when measured after the plants develop to
the mature autotrophic phase. The redox potential is determined
from exudate from the seeds.
FIG. 5 is a schematic view of an apparatus 100 with a probe
coil 101 for examining the induced-energy wave form from the
ion-electron avalanche pulses produced by the apparatus of FIG.
1A. The coil 101 had 80,000 turns of #40 copper wire and was
approximately 8 cm in diameter and 10 cm long on core 102.
The upper part of FIG. 6 is a graph showing the induced
magnetic field in the coil 101 of FIG. 5 produced by the
electron avalanches shown in the lower portion of FIG. 6. This
gives a direct reading of the current between the electrodes 11
and 12 of FIG. 1A at an applied potential of 5 kV.
FIG. 7 is a graph showing an exponential correlation
between the electron pulsed current between electrodes 11 and 12
and the magnetic field potential induced in the coil 101.
FIG. 8A is a graph showing 1995 field emergence rates in
avalanche-exposed soybeans versus two control series. The seeds
were Var. PS-202 (total of 48 seeds per test series). Series A:
5 kV, 5 min. Series B: 10 kV, 5 min. The seeds were stored for
86 days after treatment before planting.
FIG. 8B and 8C are graphs showing 1995 field emergence
rates in two varieties of avalanche exposed sweet corn seed
versus their controls. The seeds were stored for 56 days after
treatment before planting.
FIGS. 9A and 9B are graphs showing fruit or ear development
in two varieties of 1995 field-grown sweet corn versus their
controls. The seeds were stored for 56 days after treatment.
FIG. 10 is a graph showing carrot foliage yields in 1995 as
a function of avalanche-inducing voltages. The field plot data
is based on percent change in fruit relative to controls. Each
point is a mean of a series of seeds exposed at 10 sec., 30
sec., 5 min. and 30 min. at the kv level indicated. The seeds
were stored for 81 days before planting.
FIG. 11 is a circuit diagram 200 in box 20 of apparatus 10
for producing the spontaneous organized electron-ion avalanche
pulses.
FIG. 12 is a circuit diagram for a power pack nodule 201 as
shown in FIG. 11 in circuit 200 with the organized electron
avalanches used in the method of the present invention.
FIG. 13 is a connector for the power pack nodule 201 of
FIGS. 11 and 12.
FIG. 14 is a graph showing changes in avalanche pulse
amplitude as a result of photon-released electrons generated by
ultraviolet light exposure at the cathode. There is no effect
from exposing the anode, as we would expect from theoretical
considerations.
FIGS. 15, 16 and 17 are graphs showing the results of aging
of the seeds for sweet corn (G18-86), carrots, pepper and oats
with an exposure time of 25 seconds.
FIG. 18 is a graph showing the results of treating seeds in
the panicle.
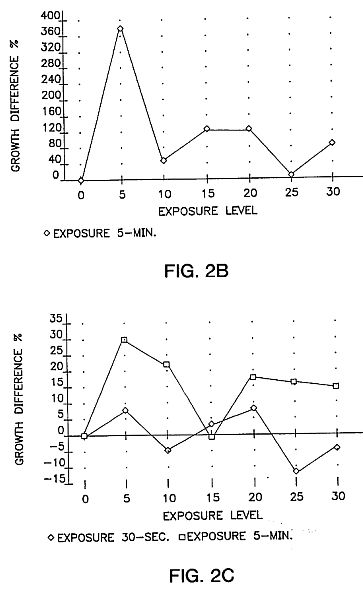
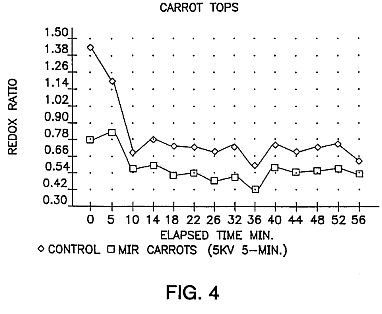

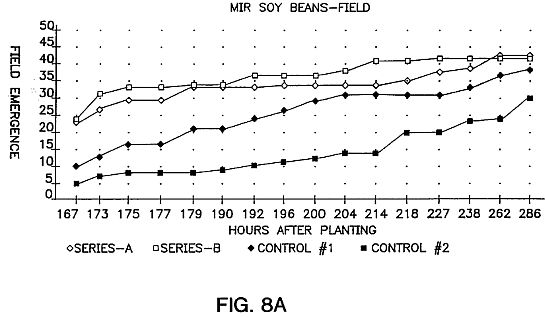
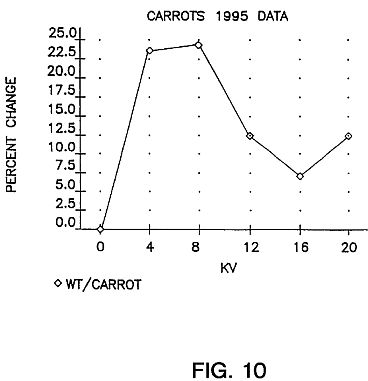
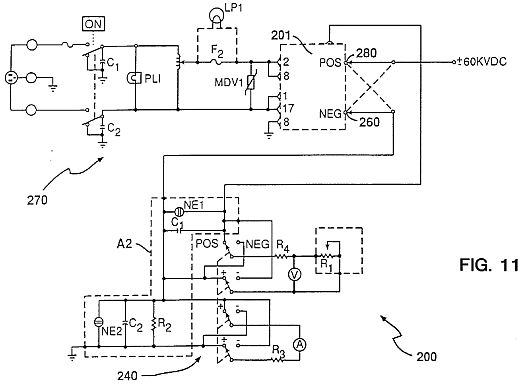
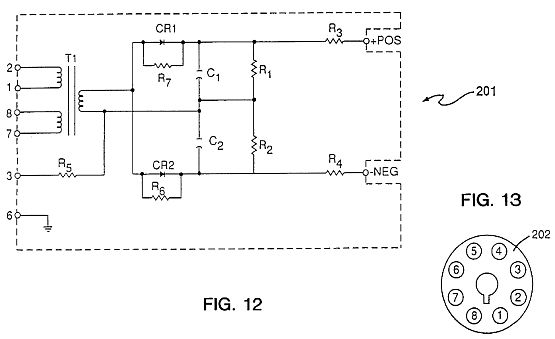
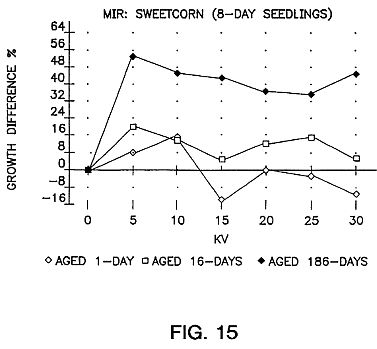
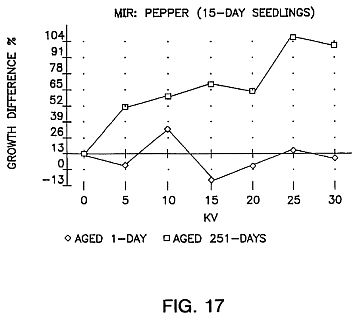
DESCRIPTION OF PREFERRED EMBODIMENTS
The present invention relates to a method for treating a seed
to enhance growth characteristics of the seed which comprises:
providing the seed between a pair of spread apart electrodes as an
anode and a cathode having a gap between them and with seed on or
adjacent to the anode; applying a direct current (DC) voltage to
the anode and the cathode using a power supply with an output
voltage with an impressed alternating current AC ripple on the
output voltage so as to produce self-organized, or pulsed
avalanches of electrons moving from the cathode towards and into
the seed between the anode and cathode or on the anode for a
period of time which enhances the growth characteristics of the
seed; and storing the seed for a period of time before planting
sufficient to allow the seed to provide the seed with the enhanced
growth characteristics.
The present invention also relates to a seed produced by providing
a space between an anode with the seed and the cathode, exposing
the seed to pulsed avalanches of electrons produced by applying a
DC voltage, with an AC ripple impressed upon the DC voltage, to
spaced apart electrodes using a power supply with an impressed AC
ripple in the output voltage to produce self-organized pulsed
avalanches of ion-electrons which move towards and into the seed,
and then storing the seed before planting.
The present invention also relates to a plant produced from a seed
produced by exposing the seed to pulsed avalanches of electrons
produced by providing spaced apart electrodes which are an anode
and a cathode with the seed between the anode and cathode or on
the anode, applying a DC voltage with impressed AC ripple to the
spaced apart electrodes to produce self-organized avalanches of
electrons which move towards the anode and into the seed before
planting.
The present invention also relates to an apparatus for detecting
the presence of pulsed avalanches of electrons in an apparatus for
treatment of a seed which comprises: a solenoid coil with multiple
turns which is adapted to be positioned adjacent to a pair of
spaced apart electrodes including an anode supporting the seed;
and detection means for detecting an induced current in the coil.
The present invention relates to an apparatus for treating a seed
to enhance the growth characteristics of the seed which comprises:
a pair of spaced apart electrodes as an anode and as a cathode
having a gap between them wherein the seed is to be supported on
or adjacent to the anode; voltage generating means for
simultaneously supplying a direct current (DC) voltage to the
anode and the cathode using a power supply with an output voltage
with an impressed alternating current AC ripple as the output
voltage so as to produce organized, pulsed avalanches of electrons
moving from the cathode towards and into the seed on the anode for
a period of time which enhances the growth characteristics of the
seed; and coil means with multiple turns mounted adjacent to the
spaced apart electrodes which detects pulsed avalanches of
electrons; and recording means for recording the pulsed avalanches
of electrons as detected by the coil means.
The present invention relates to a method for significantly
improving the rate and uniformity of germination and early growth,
as well as increased yield, in plants, particularly commercial
crops, by a cost-effective treatment of the seeds using electron
avalanches in a manner that can be reliably duplicated, and lends
itself well to commercial exploitation. The method provides an
apparatus for exposing seeds to organized avalanches of electrons
from a flat electrode.
The seeds 13 are placed directly on top of a horizontal, flat
aluminum (or other metal) plate or electrode 11 which is an anode
spaced from an electrode 12 which is a cathode so that the
electrode 11 is the bottom most of the two parallel electrodes 11
and 12. Alternatively, the seeds can be placed on a non-conducting
screen 22 (FIG. 5) elevating them above the anode electrode 11.
For all results listed here, the elelectrodes 11 and 12 used were
round and 30 cm in diameter. Other shapes and sizes of electrodes
can be used, though this may change the effective voltage levels.
The electrodes 11 and 12 are supported by legs 14 and 14A made of
a dielectric material. The bottom electrode 11 can take a variety
of forms, such as a metal conveyor belt (not shown).
A high-voltage DC power supply 20 providing positive current is
connected to the bottom electrode (anode) 11, while the top
electrode 12 (cathode) is grounded. Improved results are obtained
if the DC power supply contains an organized 60 or 220 Hertz
ripple in the DC. Other than such an AC trace and its resultant
ripple, there is no other oscillation of the DC current. This
distinguishes the apparatus from prior art systems which use a
voltage oscillator, usually in the megahertz range or higher.
Due to conductivity of the air between the electrodes 11 and 12,
organized avalanches of electrons travel from the negative
electrode 12 (cathode) to the positive electrode 11 (anode). These
electron avalanches register as pulses on the monitoring equipment
described hereinafter. When a "clean" signal DC power supply is
used, both the frequency and amplitudes of the ion-electron
avalanches are lower and more irregular. When a power supply with
AC ripple is used, the avalanches form in regular self-organized,
discrete pulses. These avalanche pulses commonly occur in the 0.1
to 30 Hz range between the electrodes 11 and 12 and are a product
of voltage gradient and conductivity of the air between the
electrodes 11 and 12, not of an artificial oscillator. The term
"self-organized" means that there is a discharge between the
electrodes 11 and 12 dependent upon the voltage and the
environmental conditions between the electrodes 11 and 12.
The best results have been obtained when the electrodes 11 and 12
are supported on dielectric legs 14 on a plastic-topped table 16
and the bottom electrode 11 is grounded to the tabletop by a
feedback loop 15 of a conductive metal. When the feedback loop 15
is added, the same electrode system produces pulses of very
similar frequency to those obtained without the loop, but of
significantly increased amplitude. The reason for this is that the
table top 16 appears to function as a feed-back loop type of
capacitor.
It has been found that an avalanche inducing voltage improving the
seeds of some plant varieties was ineffective or actually harmful
to seeds of other varieties. Likewise, the duration of the seed's
exposure to the electron avalanches is important and variable. The
diagnostic process to select the best times and voltages is also
important. Finally, the waiting period before planting, and
considerations of moisture in the air and seed temperature are
important. The present method works well on seeds dried to normal
levels for commercial storage and at temperatures above 40 DEG C.
Monitoring apparatus, described later, can be used to adjust for
altered air conductivity due to changes in relative humidity.
The method of the present invention is referred to as Molecular
Impulse Response, or MIR. A specific type of impulse from an
electron produces a molecular response in the seed which
ultimately results in significantly-improved seed performance,
when it is applied in the following manner, including but not
limited to:
A.) Electrodes and Power Supply: Using a spacing between
electrodes 11 and 12 (preferably 8 cm although other spacings,
preferably between about 1 and 20 cm, can be used but will alter
the effective voltages) and inducing a voltage gradient between
the electrodes of about 2 kV or more (other voltages can be used
up to, but below, the electrical breakdown voltage in air corona
discharge) results in the production of organized electron
avalanches which take the form of sharp, regular electrical
conductivity pulses of relatively uniform amplitude in the air
between the electrodes 11 and 12 (as traced on a chart recorder
system 21 as shown in FIG. 1A) Such spontaneously organized
electron avalanches have been described in the scientific
literature, most notably by Nasser, as examples of a low density,
low energy plasma in air at ambient pressure. (Source: E. Nasser,
"Fundamentals of Gaseous Ionization and Plasma Electronics",
Wiley-Interscience, New York, pages 209 to 217 (1971)).
The frequency of the avalanche pulses rises spontaneously with
increasing voltage (see FIG. 1B). This is different from the
oscillating electric field employed by the prior art in which the
frequency is fixed artificially and remained the same unless human
intervention changed it. This difference is at the heart of the
present invention because it is not oscillation of the electric
field which produces the desired results but these spontaneous,
organized avalanches of ion-electrons produced between the
electrodes 11 and 12 in air which elicit the Molecular Impulse
Response.
Use of a pure DC power supply, with no AC ripple, results in
electron avalanches with significantly less pulsing and
regularity. Exposure of seeds to these pulses results in a lower
seed performance than those exposed to a DC power supply with an
AC ripple. Furthermore, results are difficult to consistently
reproduce when an AC ripple is absent. Thus it is not merely
exposure to an electric field which produces the beneficial
results claimed here, nor is it exposure to any type of electron
avalanches. The seeds must be exposed to the sharp, regular,
uniform or organized electron avalanches as shown in FIG. 1B for
best results.
B.) Diagnostic Procedure: Different voltages (generally between
2-20 kV) and different time exposures (from seconds to minutes)
produce the best results with different varieties of seed. The
optimal parameters are selected for each seed by exposing them at
a range of voltages for a range of times, and comparing the
results by germination and/or growth and/or yield tests, as well
as by redox measurements.
A redox diagnostic procedure allows the achievement of significant
improvements in a wide variety of seed/plant types. This
diagnostic procedure is necessary because a variety of seed which
is positively effected at a high (20 kV) or low (5 kV) voltage may
be effected negatively by a medium (15 kV) voltage. Conversely,
seeds which do well at a low voltage may do poorly at a high
voltage and vice versa.
It has been found that the seeds should be stored at 40 DEG F. to
80 DEG F. If the temperature is too low then no result is
achieved.
It will be appreciated that the seeds can be positioned on a
non-conductive screen 22, such as fiberglass, between the
electrodes 11 and 12 as shown in FIG. 5. Preferably the electrodes
11 and 12 are round with rounded edges. The electrode preferably
has a 8 to 9 cm gap and a diameter of about 30.5 cm. The seeds are
placed on the electrode so as not to be touching significantly.
EXAMPLE 1
This Example shows laboratory germination tests accurately
diagnosing treatment levels which produce yield increases, plus
examples of how a voltage which is good for one crop produces
marginal or decreased yield in another, as compared to untreated
controls as shown in Table
TABLE 1
Best Germ. Good Yield@1
Marginal or
Crop Type kV kV Poor Yield
Tomato 5 kV 4, 12, 16 kV
8, 20 kV
Carrot 5 kV 4 kV 12, 20 kV
Soybeans 8 kV 8,12 kV 4 kV
Navy Beans
10 kV 10, 12 kV 6 kV
Bi-Color 15 kV 16, 8 kV 12, 4 kV
Sweet Corn
Kandy-Krisp
15 kV 16, 12 kV 4, 8 kV
Sweet Corn
Inbred 4, 16 kV 8, 12, 20 kV
Field Corn
Hybrid 4, 12, 16 kV
8, 20 kV
Field Corn
Cypress 15 kV 16 kV
Rice
@1 Measured by fruit and grain weights.
Frequently, laboratory germination voltages were tried in
increments of 5, i.e. 5, 10,. 15 kilovolts, while field tests were
in increments of four kilovolts, thus producing non-exact matches.
Results of a range of treatment durations have been averaged here
for each voltage for simplicity.
A key element of the present invention is a waiting period during
which treated seeds are not germinated for a minimum of several
weeks after exposure. Germination of exposed seeds before this
waiting period is completed can result in no improvement in the
seeds or even negative effects. Consistent, reproducible,
improvements are not found with seeds planted soon after exposure.
Improved effects in treated seeds have been seen as long as 18
months after treatment. There is not as yet any known upper limit
to the waiting period. While the minimum waiting period varies
from one seed variety to another, a minimum of 30 days has been
found to be effective. The seeds of FIGS. 2A to 2C were stored for
35, 35 and 36 days respectively.
The redox ratio is a measure of temporal variations in respiration
as measured by changes in oxidation/reduction activity in
seedlings grown from treated seeds. Increased phase amplitudes of
redox cycles, indicative of increased rates of respiration and
free radical activity, have been consistently measured in 10-12
day seedings grown from MIR-treated seeds (FIGS. 3A and 3B). Many
studies have suggested that alterations in redox ratios are linked
with growth responses in biological organisms. (Levengood,
"Bioelectrochemistry And Bloenergetics, 19 461-476 (1988); also
Allen and Balin, "Free Radical Biology and Medicine" Vol. 6, pp.
631-661 (1989); A. Sakamoto et al., FEBS Letters, Vol. 358 pp. 62
(1995)). Whether or not this is in fact the mechanism of the
present invention, alterations in redox ratios have been seen to
be linked with improved growth performance in MIR-treated seeds,
including eventual increases in final yield. In the green seedling
autotrophic stage, redox levels of seedlings grown from
MIR-treated seeds are lower than in untreated seedings as shown in
FIG. 4, consistent with the hypothesis of higher levels of
anti-oxidants present which deactivate free radicals and thereby
lower redox ratio levels.
Measurements were made according to the procedure set forth in
Levengood, Bioelectrochemistry And Bioenergetics, 19 461-476
(1988). Detection of the above-mentioned free radical alterations
can be used as a means of quality control for MIR operations. This
monitoring or quality control can serve as a rapid check that the
desired effect is being achieved in the treated seeds, without
resorting to time-consuming growing of the seeds. This redox ratio
analysis makes commercial scale operations reliable and
dependable.
From several hours to several days after treatment, MIR seedlings
display raised redox ratios, indicating a burst of free radicals
within the cells formed by the impact of the ion-electron
avalanches. Seeds experience activation of cellular anti-oxidant
defenses and consequently have lowered redox ratios. In dried
seeds this process moves slowly, as do all metabolic processes in
quiescent seeds. Seeds which have been treated at an effective
voltage and for an effective time will, during storage, experience
a redox level shift as cellular anti-oxidant defenses, such as
Superoxide Dismutase (SOD) and others, deactivate the free
radicals. In maize, for example, cells have been known to produce
more SOD than needed to disable the free radicals present. Gail L.
Matters and John G. Scandalios, "Effect of the free
radical-generating herbicide paraquat on the expression of the
superoxide dismutase (Sod) genes in maize", Biochemica et
Biophysica Acta 882 p. 33 (1986) observed 54% increases in SOD
levels but only a 40% increase in SOD activity, in response to a
burst of superoxide radicals. Thus the resulting surplus of
anti-oxidants lowers the normal levels of free radicals in seeds
and in mature, developing plant tissue the MIR treated plants have
lower redox ratio than in the untreated controls as shown in FIG.
4.
As shown in FIG. 5, the spatial drift of the MIR pulses outside
the electrodes 11 and 12 can be examined by stationing an
experimental probe coil 101 near the electrodes 11 and 12. A
linear chart recorder 21 is used to detect the induced current in
coil 101. The electron avalanches drift laterally from between the
electrodes 11 and 12 and through an electrostatic-magnetic
coupling induce a magnetic field in the coil 101, which in turn
generates a potential in the millivolt range. With the coil 101
placed directly across one channel of a dual channel chart
recorder such as recorder 21 in FIG. 1A and the MIR system across
the second channel, one can examine the effectiveness and form of
the pulses in action. For example, the set of curves in FIG. 6
show the magnetically induced and MIR pulses from the coupled
system. The coil 101 usually has 10,000 to 100,000 turns,
preferably 80,000 turns.
As pointed out by H. Raether ("Electron Avalanches and Breakdown
in Gasses", Butterworth & Co. Ltd., U.K. 1964) one reliable
criteria to know whether an observed current pulse can be
identified with an avalanche process is to compare the form of the
avalanche pulse with the induced magnetic component. From the
basic theory of electron avalanche formation one should find that
the induced magnetic component H (expressed here as coil 101
potential) is directly related to ln(i), where i is the amplitude
of the avalanche current pulse in the MIR system. The experimental
data in FIG. 7 confirms (r=0.89; P<0.05) that these are
electron avalanches.
EXAMPLE 2
When the above steps are used together as part of a coherent
process to treat the seeds in the aforementioned manner, the
following results have been achieved in a variety of crops in both
laboratory and field tests:
1) Increased rate of field emergence. An example is shown in FIG.
8A for Glycine max. Var. PS-202 and in FIGS. 8B and 8C for two
varieties of Zea mays sweet corn.
2) Increased rates of plant growth and plant size uniformity.
EXAMPLES 3 AND 4
Examples of the MIR effect in sweet corn are disclosed in Table 2
and 3 below. The data were taken at 52 days development within
field test plots. The seeds were stored for 56 days.
Variety-"Kandy
TABLE 2
Plant heights (cm)
N- Coeff.
kV-
Series ave. sd plants of Var.
level
Controls 113.2 29.8 49 26.3% None
5 sec. 145.2 11.3 31 7.8% 12-16
10 sec. 134.8 26.7 37 19.8% 12-16
Variety
TABLE 3
Plant heights (cm)
N- Coeff.
kV-
Series ave. sd plants of Var.
level
Controls 109.6 36.3 81 33.1% None
5 & 10 126.6 28.4 43 22.4% 12-16 sec.
5 min. 123.2 28.4 36 23.1% 12-16
EXAMPLE 5
Increased lateral root growth which has been achieved.
Navy bean seed were treated on Sep. 30, 1992 and germinated 65
days later (20 seeds per lot) as shown in Table
TABLE 4
3 Day
Voltage Duration Roots sd Number
5kV 25 sec. 6.26 cm 1.64 20
10 kV 25 sec. 6.63 cm 0.92 19
Control 0 4.54 cm 2.63 20
EXAMPLE 6
Accelerated maturity has been achieved. Some plants grown under
open field conditions from treated seed reach the harvest stage in
significantly fewer days, as compared to controls. With sweet corn
of two varieties, ears with protruding silk were counted 52 days
after they were planted as shown in FIGS. 9A and 9B.
EXAMPLES 7, 8, 9, 10, 11, 12
Increased Yield has been achieved in a variety of commercial crops
under normal field conditions, with no extraordinary use of
sprays, irrigation, or fertilizer. These effects have been noted
in various plants. Soybeans: with a +28.6% increase in yield by
dry weight of Soybean seed (Glycine max) of variety 05-202, were
exposed for 5 minutes to voltages of 5, 10, 20 and 30 kV on Mar.
2, 1994. One row of 48 seeds from each of these series was planted
May 27, 1994 (25 days later) in individual field test plot.
Emergence was noted as shown in FIG. 8A, with significant
improvements over controls. The best emergence was seen in the 5
kV and 10 kV exposures. These two exposures were the same ones
which showed increases in yield at harvest. The results are shown
in Table
TABLE 5
Series Voltage Yield in Lbs.
Control Controls 1.75 lbs.
A 5 kV 2.25 lbs.
B 10 kV 2.20 lbs.
D 20 kV 1.63 lbs.
E 30 kV 1.50 lbs.
Soybeans: In a 1995 field test, seeds of Soybean var. "Young" were
treated Mar. 15, 1995 and planted May 12, 1995. Each field plot
entry represents the mean of four replicates from a two pound lot
of treated seed. Results were converted to bushels per acre.
Weights per 1,000 seeds from harvest showed appreciable
differences. Yield increases were the result of more soybeans
produced. The results are shown in Table
TABLE 6
TREATMENT BUSHELS/ACRE
Control 35.95
4 kV, 10 sec. 37.04
4 kV, 30 sec. 34.99
4 kV, 5 min. 36.04
8 kV, 10 sec. 40.10
8 kV, 30 sec. 41.44
8 kV, 5 min. 41.73
12 kV, 10 sec.
34.74
12 kV, 30 sec.
39.50
12 kV, 5 min. 39.64
Control 34.92
Field Corn: 24 seeds per lot were planted on May 31, 1995 in
Blissfield, Mich. Figures are pounds of shelled corn per lot. The
results are shown in Table
TABLE 7
Inbred, Variety 305-10Gr (F6)
VOLTAGE 10 sec. 30 sec. 5 min.
Control
4 kV 2.65 lbs.
1.85 1.55 2.10
8 kV 1.80 1.95 1.45 1.95
12 kV 1.95 1.35 1.50 1.90
16 kV 1.60 1.00 0.95 2.00
Mean of Controls: 2.03
Hybrid, Variety HYPOP.2830MF. The results are shown in Table
TABLE 8
VOLTAGE 10 sec. 30 sec. 5 min.
Control
4 kV 7.15 lbs
7.10 6.65 5.55
8 kV 5.05 4.40 4.75 4.90
12 kV 5.95 5.65 4.85 4.20
16 kV 5.20 5.95 5.10 6.10
20 kV 5.20 4.75 3.95 3.20
Mean of Controls: 4.79
Carrots: Carrot seeds of variety Daucus carota Danvers 126 were
planted May 31 1995 at Blissfield, Mich. and harvested Sep. 7,
1995. Weight per carrot figures are summarized by voltage in FIG.
10. Below are results per treatment duration for 4 kV and 8 kV
(best yielding voltages) plus controls. In these results the
interplay and dual importance of both time and voltage level is
obvious. Here the increases over controls follow no linear
progression, emphasizing the importance of the diagnostic
procedures discussed earlier in order to select the most effective
voltage and treatment duration for a particular seed variety. The
results are shown in Table
TABLE 9
VOLTAGE DURATION WT./CARROT
4 kV 10 sec. / 0.10 lbs.
4 kV 30 sec. / 0.112
4 kV 5 min. / 0.141
4 kV 30 min. / 0.128
8 kV 10 sec. / 0.066 lbs.
8 kV 30 sec / 0.154
8 kV 5 min. / 0.175
8 kV 30 min. / 0.093
0 0 0.10 lbs-- /Control
0 0 0.096-- /Control
0 0 0.105-- / Control
0 0 0.089-- / Control
0.098 Mean of Controls
Tomatoes: Seeds of Lycopersicon esculentum variety malinta were
exposed Mar. 10, 1995 and planted May 31, at Blissfield, Mich. and
harvested Sep. 5, 1995. Yield in pounds of fruit per plant was
averaged for each voltage across four time exposures (10 sec., 30
sec. 5 min., and 30 min). The results are shown in Table
TABLE 10
VOLTAGE LBS./PLANT % CHANGE
Control 0.516 / 0%
4 kV 0.69 / +34%
8 kV 0.455 / -12%
12 kV 0.648 / +26%
16 kV 0.61 / +18%
20 kV 0.458 / -11%
Rice: Cypress rice (Oxyza sativa) seed of variety Lemont was
obtained from Mississippi State University, treated Mar. 12, 1995,
and planted May 11, 1995 (59 days) in Mississippi. Test plots were
flushed with water May 15 due to extreme dryness. Emergence
occurred May 25 (delayed due to dryness) and plots were flooded
June 9. Each figure is the result of 250 gms. of seed grown in
four replicated plots, averaged and extrapolated to bushels per
acre. Peak yield increases were noted as shown in Table
TABLE 11
VOLTAGE TIME YIELD % CHANGE
Control 0 159.37 / 0%
16 kV 10 sec. 180.13 / +13%
16 kV 30 sec. 169.06 / +6%
8 kV 5 min. 170.08 / +7%
FIGS. 11, 12 and 13 show the circuit 200 of the apparatus of the
present invention. The apparatus is available from Hipotronics,
Inc., Brewster, N.Y. There is an AC circuit 220 and a DC circuit
240. The negative terminal 260 is connected to the cathode
electrode 12 and the positive terminal 280 is connected to the
anode electrode 11. The various elements in the apparatus of FIG.
11 are shown in Table
TABLE 12
220 Circuit
C1 .022 600 V
C2 .022 600 V
PLI
F2 2A
UP1
MDV1 250 V
200 Circuit
NE1
NE2
POS Positive
NEG Negative
R1 5 K 1/4 W
R2 5 K 1%
R3 250 K 1%
R4 270 K
A2 Meter Circuit P/N 30-293
C1 .22 400 V
C2 .22 400 V
201 Circuit
T1 Transformer
R1 250 M, 6 W
R2 250 M, 6 W
R3 50 K, 50 W
R4 50 K, 50 W
R5 200 M, 6 W
R6 22 M, 1 W
R7 22 M, 1 W
CR1 Diode
CR2 Diode
C1 0.02 .mu.f; 30 kV
C2 0.02 .mu.f; 30 kV
POS Positive
NEG Negative
Output 60 kV DC
2.5 mADC
FIGS. 15, 16 and 17 show the results of aging of the seeds for a
period of time. As can be seen the aging is very important.
FIG. 18 shows the results when oat seeds are treated in the
panicle which tends to shield the seed from the electrons. As can
be seen, the treatment is effective but less so than in FIG. 17.
It is believed that the influence of the MIR process on seeds is
based on the formation of electron-ion avalanches in air at normal
atmospheric pressure and temperature. Under an applied electric
potential, these avalanches can be directed as electron-ion
impulses in the form of regular cycles or plasma waves. The
frequency, amplitude and confinement of these pulses are governed
by the applied potential and the design configurations of the MIR
apparatus.
In the MIR process there is a relationship between the
electron-ion avalanche pulse formation and the manner in which
they form an organized plasma. The avalanche formation takes place
between parallel plate electrodes 11 and 12 at a potential
sufficient to cause the electrons (e@-) leaving the cathode to
gain enough energy to ionize air molecules through both elastic,
and to a lesser degree, inelastic collisions. In the present MIR
configuration the minimum potential for avalanche formation is
around 0.5 KV/cm. In the electron-molecule collisions new e@- 's
are formed and these plus the primary e@- keep repeating this
process thus forming a cascading avalanche.
The mean number (n) of drifting electrons e@- 's grow at,
n(x)=exp (.alpha.x) (1)
wherein x is the distance of e@- drift, and .alpha. the mean
number of ionizing collisions per e@- per cm. Nasser (E. Nasser,
Fundamentals of Gaseous Ionization and Plasma Electronics,
Wiley-Interscience, New York (1971)) points out that after a time
t' the electric field disappears within the avalanche so that the
e@- swarm stops and attaches to molecules, that is, the plasma
pulse is partially neutralized or discharged. This takes place
inside the electrode gap if the drift path L of the avalanche is,
L=vt' (2)
wherein v, the e@- drift velocity is less than the electrode
spacing distance d (in air, v is around 10@7 cm/sec.). With d=8
cm, t' must be <8.times.10@-7 sec. The positive ions (not shown
in FIG. 1A) have a low v@+ of around 10@5 cm/sec and therefore
have drifted very little from their point of production.
The current i produced by an avalanche is,
i=(.epsilon.n0 /t')exp (.alpha.v't) (3)
If we take (.epsilon. n0 /t') as the rate constant k', for the
avalanche formation,
i=k'exp (.alpha.v'T) (4)
where T is the transient time for one avalanche pulse, therefore
ln(i)=k(.alpha.v'T) (5)
wherein k is a new rate constant. Thus in (i) is proportional to
the mean number of ionizing collisions (.alpha.) during an
avalanche pulse of transient time T.
One reliable criteria (H. Raether, Electron Avalanches and
Breakdown in Gasses Butterworth & Co., Ltd., Great Britain
(1964)) to know whether an observed current pulse can be
identified with an avalanche process is to measure and compare the
growth of e@- 's with the theoretical relationship.
n=exp (.alpha.v t) (6)
In the MIR system there is no e@- confinement, therefore the
avalanche pulses drift laterally outside the confines of the
parallel plate electrodes. This external drift of plasma provides
a method for experimentally examining the growth of electrons as
predicted by the Equation-6 theoretical relationship. For this
purpose an experimental probe coil 101 consisting of 80,000 turns
of #40 copper wire, was positioned in proximity with the MIR
system (FIG. 5). When placed directly across one channel of a
linear chart recorder, any induced magnetic field is readily
detected as a voltage pulse in the probe coil 101. Avalanche
pulses of varying current amplitudes were formed within the MIR
system and recorded on a separate recorder channel as shown in
FIG. 6. Any induced field in the probe coil is taken as being
proportional to the plasma density formed by the ionizing
collisions. From Equation 5 the predicted relationship between a
transient avalanche current s and the magnetic field H, induced by
an ion-electron concentration (.alpha.) drifting across the test
coil 101 would, under these hypothetical conditions be given by,
H=c1 ln(i)+c2 (7)
wherein c1 and c2 are proportionality constants.
From chart recorder traces taken from experiments conducted over a
range of electrode potentials, the amplitudes (in mv) of the
plasma induced magnetic fields were compared with the amplitudes
of the avalanche currents. These data (FIG. 7) plotted according
to Equation 7 show good correlation (r=0.89; P<0.05) between
the theoretical model of plasma avalanches and the experimental
data obtained from the MIR system.
At a given potential the amplitudes and frequency of the avalanche
pulses remain relatively constant over the transient intervals.
The stability of the ion current pulses was examined by
"injecting" excess electrons into an MIR system during a
succession of stable avalanche pulses. If UV radiation is directed
onto the cathode plate, electrons are released through the
photoelectric effect. This can produce what has been called (H.
Raether, Electron Avalanches and Breakdown in Gasses, Butterworth
& Co., Ltd., Great Britain (1964)) "Avalanches With
Successors". Through the injection of additional secondary
electrons the amplitudes of the avalanche pulse currents are
increased.
This photoelectric avalanche enhancement was produced in a MIR.
system consisting of "Optical Transmitting Electrodes" or OTE's
(glass coated with a semiconducting tin oxide film) as electrode
12 arranged with electrode separation of 6 cm and 20 kV applied
potential. As shown in FIG. 14, the effect of the electron
injection is shown to take place 30 seconds after the start
(indicated by arrow) of cathode exposure. Due to a shielding
effect (E. Nasser, Fundamentals of Gaseous Ionization and Plasma
Electronics, Wiley-Interscience, New York (1971)), a plasma will
tend to remain stable even when external charges are introduced
into the avalanche system. This initial delay followed by a rise
to a maximum current amplitude at around 70 sec. followed by the
gradual decline, is very consistent with the results obtained in
other plasma systems, again confirming that it is a plasma
electron avalanche process at work in the space between the
electrodes. Exposure of the anode (polarity reversed) to UV had no
effect (lower curve) on the current pulse amplitudes, as would be
expected. using an anode which is wider than the cathode alters
the shape of the electric field in a manner which contains more of
the ion/electrons between the electrodes, allowing fewer to drift
outside. The result is even more uniform and regular pulses of
ion/electron avalanches.
The commercial advantages of the present invention are:
(1) Germination and Early Growth: With the MIR method the plant
moves through the vulnerable, seedling stage faster. Greater
uniformity at this stage limits the disadvantages of taller plants
shading shorter ones and increases chances for all to thrive.
Uniformity of growth also makes it easier to harvest the plants.
(2) Root Growth: The MIR method is of particular value in plants
such as navy beans where root growth is frequently a problem.
(3) Accelerated Maturity: Accelerated maturity due to the MIR
method is of economic advantage to farmers in crops, such as
tomato and sweet corn, where the first produce to market each
season commands much higher prices. In countries which double
crop, it increases the likelihood that both crops will be able to
mature and produce a full harvest. In far northern regions, with
limited daylight and warm days in growing season, the MIR method
increases the chances of a successful season.
(4) Increased Yield: There are economic and humanitarian
advantages to the MIR method. There is commercial appeal to the
farmer, allowing him to grow more crop to produce income from the
same farm. With world population growth outstripping food supply,
any significant increases in yield is beneficial.
Key features of the MIR method are:
(1) Sharp, well-organized, uniform electron avalanches (not corona
discharge, and not static electric fields). This is provided with
a DC voltage source having an AC ripple.
(2) Voltage potentials are 0.2 vK/cm to (but not including)
dielectric spark gap breakdown discharge.
(3) Anode electrode with the seeds.
(4) Special electron feedback loop 15 enhances results.
(5) Diagnostic Procedures.
(6) A waiting period of several weeks between treatment and
planting.
(7) Redox ratio measurement provides quality control after
treatment by the MIR method to confirm if effect was achieved,
thus providing an immediate check on results.
(8) Coil 101 recorder system provides an additional quality
control to insure avalanches are in fact being produced, and have
the proper form. Without this test, humidity and dust/debris on
electrodes 11 and/or 12 could cause failure to produce avalanches
(particularly when operating near the 0.5 kV/cm threshold, which
is frequently used with some seeds.
(9) The MIR method is practical and affordable for large scale
commercial operations. Short time period of treatments are
required (seconds to minutes) and small amounts of electricity are
expended. The MIR method is suitable for conveyor-driven seed
handling systems. The MIR method produces consistency of results.
It is intended that the foregoing description be only illustrative
of the present invention and that the present invention be limited
only by the hereinafter appended claims.
Method for Determining the Viability
of Seeds Prior to Planting
CA1003496
[ PDF ]
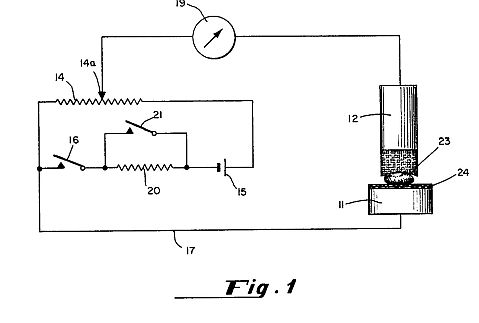
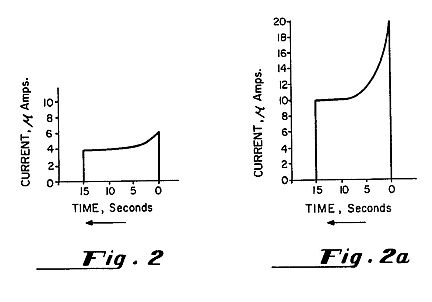
Seed Science and Technology (Netherlands) v. 9(2) p. 567-576
(1981)
Steere, W.C., Levengood, W.C., Bondie, J.M.,
Agro Sciences Inc., Ann Arbor, Mich. (USA),
An electronic analyser for evaluating
seed germination and vigour.
The electrical currents conducted through exudate solutions from
100 individual seeds were measured with a Model ASA-610 Seed
Analyser and compared to standard laboratory germination and
certain aspects of seed vigour. Examination of the histograms of
current distribution from 100 seeds showed a shift towards higher
current ranges as seed lot quality declined. A simple technique of
data analysis of the current levels provided a "germination
prediction" which correlated well with standard laboratory
germination. Results from various commercial lots of soybeans
(Glycine max), bushbeans (Phaseolus vulgaris), and cottonseed
(Gossypium hirsutum) showed that the method has broad potential
usefulness as a rapid indicator of commercial seed lot quality.
Method for producing new varieties of
plants
US5288626
[ PDF ]
A method for increasing the proportion of mutants in a generation
in a first plant species having a recognized and established
phenotype involves the simultaneous somatic exposure of germinal
plants of the species to contact with whole cells and associated
material of a second species of plants, and to electrophoretic
conditions. The plants of the first species are preferably in a
germinal state, such as seeds or seedlings, while the whole cells
and associated materials of the second species can be a seedling
root tip, a seedling, a tissue macerate (suspended in either water
or agar) root nodules, fruit tissue or root tissue. When the cells
of the first and second species have different membrane
potentials, the step of electrophoretic exposure can be carried
out by simply placing the cells in contact with one another.
Preferably, however, an electropotential difference such as a
constant DC voltage is disposed across the somatic cells of the
first species of the plant and the whole cells and associated
materials of the second species of plant, for example, by
attaching one of a cathode and anode to the first species of
plant, and the other of anode or cathode to the second species of
plant.
BACKGROUND OF THE INVENTION
1. Field of the Invention
The present invention relates to a method for inducing mutations
in plants, and more particularly to a method for increasing the
number of plants of a first species which exhibit a phenotype or
characteristic normally associated with a different species.
2. Description of the Prior Art
The members of a given species of plant typically share a number
of well-established physical characteristics associated with the
genetic materials of their cells; these characteristics are known
as phenotypes. However, it is well known that plants of a given
species having one or more new and distinctive characteristics,
generally referred to as sports or mutations, occur naturally as a
small fraction of any plant population. For centuries, mutants
have been selectively bred to produce new varieties or
modifications of existing plants. In natural populations of
plants, however, the frequency of mutations is generally
considered to be less than 1 in 500,000, so that the selection of
desirable mutants after such breeding is a slow and laborious
process, particularly since it is well recognized that mutants
exhibiting a desirable phenotype are rare, and progeny outputs are
often low.
Several methods for increasing the occurrence of mutants in a
population of a given species are well known; for example, the
exposure of such a population to ionizing radiation. Such
techniques, however, are typically subject to the drawbacks that
the individually resulting mutants are generally weak, and must
still be subjected to the time-consuming and labor-intensive
techniques of isolation and selective breeding for a large number
of generations, before a sufficient number of mutants possessing
the new phenotype are obtained for use in outcrossing or
agricultural growth.
Recombinant DNA and protoplast fusion techniques are potentially
useful for producing new varieties of plants without isolation of
mutants or selective breeding. The use of these techniques is
subject to several drawbacks, however. First, these techniques are
tedious and slow, requiring elaborate instrumentation involving a
large number of chemical processes, and a substantial investment
in the education and training of the personnel conducting the
procedures. Presently, these techniques are very expensive and
time consuming. Indeed, Applicant is aware of no reported instance
of the inducement of a functional expression of a novel gene
(phenotype) from one species of plant to a population of another
species of plant, employing these genetic engineering techniques.
SUMMARY OF THE PRESENT INVENTION
The present invention overcomes these and other difficulties
encountered in prior methods of inducing mutations in a population
of a first species of plant by providing a method for increasing
the number of mutants exhibiting altered phenotypic
characteristics, characteristics which are stable in successive
generations, where such phenotypic characteristics are an
established trait of a second different species of plant. The
method of the present invention allows for the production of large
numbers of plants having substantial modifications from the parent
generation, without the delay of several generations for selective
breeding and establishment of characteristics as stable by
outcrossing, and which does not require the complex
instrumentation or large numbers of chemical reactants and steps
inherent in present recombinant DNA or protoplast fusion
techniques.
The method according to the present invention involves placing a
plurality of germinal plants of a first or recipient species, this
first species exhibiting at least one established phenotype, in
contact with the whole cells and associated materials of a second
species of plant, while exposing the germinal plants of the first
species to electrophoretic conditions, such as an ionophoretic
current. The germinal plants are grown to adult plants, or to a
stage sufficient to observe any changes from the established
phenotype. The exposure of the germinal plants of the first
species to electrophoretic conditions can be carried out by simply
abutting a portion of seedlings of the first species with
seedlings of a second plant species, when the cells of the first
and second species have differing membrane potentials. This can be
carried out by excising complimentary sections from the root of
seedlings of the first and second plant species, and abutting the
cut surfaces of the roots. Preferably, however, an external DC
current is applied across the germinal first species plants and
whole cells and associated materials of the second plant species
by attaching an anode to the plants or materials of one species,
and a cathode to the plants or cells of the other species.
Typically the plants and materials are exposed to a constant DC
voltage having a current density in the range of 10 to 100
microamps per centimeters applied at a potential difference of
from 1 to 50 volts for periods of five minutes to 24 hours. In
effect, the donor material of the second species acts as an
electrode substrate or base contactable with the seedlings of the
first species. The donor material is prepared as either a tissue
macerate or as whole tissue. The donor material can be placed on
sterile cotton or a filter paper which in turn rest on a stainless
steel plate electrode. Most preferably, the acceptor tissue or
plants of the first species are exposed at the seed or early
seedling stage, typically 24 to 96 hours after germination by
placing the root apex in contact with the donor-coated electrode,
and the shoot apex, cotyledons or coleoptile in contact with the
electrode of opposite polarity.
The method of the present invention is preferably carried out with
genetically pure, stable and homozygous inbred varieties of lines
as the host or acceptor first species. Such well-established lines
were used in all of the examples described below, and are
commercial varieties which have been released from university or
USDA breeding programs for public use.
After exposure, the test seedlings or germinal plants of the first
species, along with untreated controls, are developed to maturity
under field conditions or in a greenhouse, depending upon
expediency. Typically, alterations are observed in the growth
rates and yields of the germinal plants actually treated,
depending upon the type of donor and the exposure parameters;
however, a stable expression of an altered phenotype is typically
not seen until at least the second generation bred from the
treated plants. The frequency of inherited, varietal alterations
resulting from the present method ranges from 5% to 95% of the
test population, typically, depending upon the specific procedure
and plant species involved. This is a substantial improvement over
the proportion of one in a few thousands or several thousands of
cells or plants treated by recombinant DNA and protoplast fusion
methods.
Not only does the present method yield a significantly increased
proportion of mutants in the treated plants, but a significant
proportion of the resulting mutants exhibit an altered phenotypic
characteristic which was, in fact, an established phenotypic
characteristic of the second or donor species of plant. It is
believed that this transferred phenotype results from the
transduction of genetically associated cell tissue components and
macromolecular complexes from the second or donor species into the
intact, somatic cells of the first or acceptor species, in such a
manner as to alter the genotype and/or phenotype of the plants of
the first species. For this reason, plants treated in accordance
with the method of the present invention, or grown from plants
treated in accordance with the present invention, are designated
by generation with the letter "T". For example, the first treated
generation of the first species of plant is described as the T-1
generation, while a second inbred generation grown from the adult
plants of the T-1 generation are referred to as the T-2
generation. This designation of generations is intended to avoid
confusion with the system of F-1, F-2 and so on, normally employed
in conventional plant breeding, when crossing for hybrid vigor.
It is thus an object of this invention to provide a method, by
means of electrophoresis techniques, for the production of new
plant mutations consisting of types and varieties having altered
genotypic and/or phenotypic characteristics, that is simple when
compared with the recombinant DNA and protoplast fusion methods
known in the art. The methods of the present invention do not
require complex instrumentation, nor drastic alterations in cell
wall-membrane contiguity, particularly the removal of the cell
wall as required by prior techniques, or detailed elucidation of
chromosome maps.
Another object of the present invention is to provide a method for
the production of new varieties of plants that can quickly yield
large numbers of healthy plants having substantial modifications
from the parent plants, thus eliminating the delay of several
generations and large test populations required in prior selective
breeding programs, which have been conventionally necessary before
the plants can be used in out-crossing. Both conventional breeding
programs and the recombinant DNA and protoplast fusion methods
generally produce a low yield of mutants which must be selectively
grown and bred for a large number of generations, before a
sufficient number of stable plants are available for use in
programs for developing plant varieties; in contrast, the
production of such stable plant varieties is remarkably more rapid
in the present invention.
BRIEF DESCRIPTION OF THE DRAWING
A better understanding of the present invention will now be
had upon reference to the following detailed description, when
read in conjunction with the accompanying drawing, wherein like
reference characters refer to like parts throughout the several
views, and in which:
FIG. 1 depicts a joined pair of seedlings of two species of
plant;
FIG. 2 depicts a joined pair of seedling with root tips
excised and electrodes inserted;
FIG. 3 depicts an electrical potential applied to a
seedling in contact with a treated medium;
FIG. 4 depicts the application of an electrical potential
to a liquor derived by macerating plant tissue;
FIG. 5 depicts fractionation of electrode solutions
surrounding electrodes;
FIG. 6 depicts the application of an electrical potential
across a seedlings and solution filled syringe;
FIG. 7 depicts the application of an electrical potential
across a seedling disposed on a treated filter paper;
FIG. 8 depicts a seed disposed between two pieces of
treated filter paper with an applied electrical potential;
FIG. 9 depicts the application of an electrical potential
across a single cell and a donor medium;
FIG. 10A is a graphic representation of a homeostatic
pathway model of the changes induced in plants of a first
species by the method of the present invention; and
FIG. 10B is a graphic representation of the response of the
concentration of a hypothetical metabolite when a homologous
metabolite from a different plant species is introduced into the
cell when the method of the present invention is carried out.
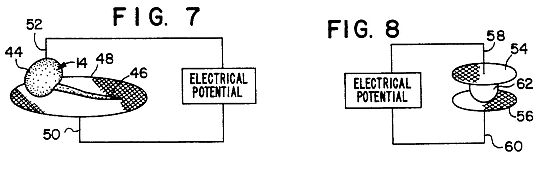
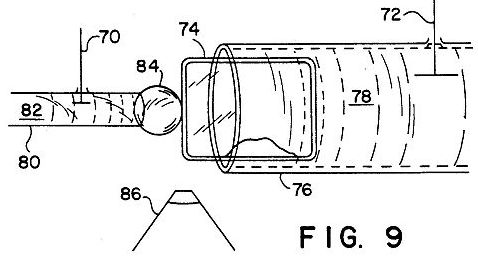
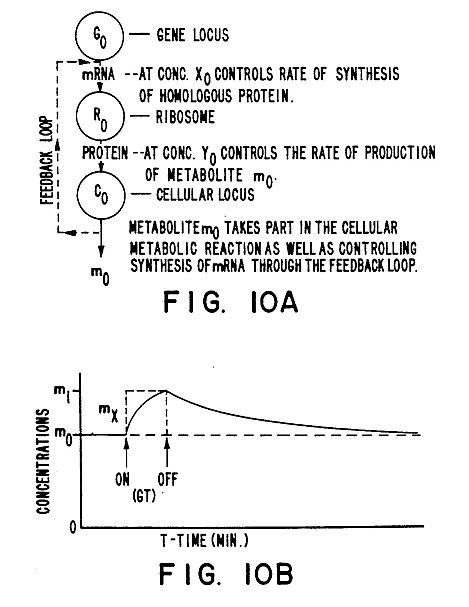
DETAILED DESCRIPTION OF THE PREFERRED EMBODIMENTS OF THE
PRESENT INVENTION
The method of the present invention both increases the proportion
of mutants in a generation of a first species of plant (the
species having at least one established phenotype) while
simultaneously causing at least some of the resultant mutant
plants to exhibit a phenotype, characteristic or trait of a second
species of plant. Several illustrative techniques and specific
examples of the present invention are described hereinafter. It
should be understood that the technique of the present invention
is generally intended to be used on a substantial number of plants
sought to be modified, so as to provide a ready supply of mutant
plants for subsequent varietal development. The electrophoretic
techniques described however, can also clearly be conducted on a
single plant cell as the acceptor, employing micromanipulative
techniques in order to apply an ionophoretic current across the
single acceptor cell and donor material. Such a technique is, of
course, within the scope of the present invention. In such a case,
the acceptor electrode can comprise a thin needle or wire inserted
into or in contact with the acceptor cell. In all cases, however,
the cell wall and plasmalemma are preferably not breached by the
present method.
It is believed that germinal plants, such as seeds or seedlings
about one to five days after germinations, are most susceptible to
successful treatment by the method of the present invention. While
some variation may occur in the percentage of mutant plants grown
from the treated seeds or seedlings, such as percentage varying
with the species of plants used and the particular technique
employed, the method of the present invention will generally
result in a substantially greater percentage of mutations than
results when radiation or the like are employed to create mutant
plants. Moreover, whereas radiation and the like cause random
mutations having widely varying characteristics, in general a
substantial percentage of mutants formed by this invention tend to
exhibit substantially similar characteristics.
With reference first to FIG. 1, a first embodiment of the method
of the present invention is thereshown involving a joined pair of
seedlings 10 of two different species of plants. One or more
seedlings 12 of first species of plant are prepared by
longitudinally excising a section consisting of about one-half of
the root's side, to expose a fresh cut surface 16. Preferably, the
germinated seedlings include radicles in the range of 1 to 6
centimeters in length, and the radicle tip is left intact when the
surface is cut, exposing the procambium, protophleom and
protoxylem cells. The root side and tip of a corresponding number
of seedlings 14 of a second, different species of plant are
excised, the radicle tip as well as the longitudinal portion of
the side being removed, to form a cut surface 18. The seedlings 14
of the second species are preferably of similar radicle
development as the seedlings 12 of the first species, and the
procambium, protophloem and protoxylem of the seedlings 14 of the
second species similarly form the cut surface 18.
The cut surfaces 16 and 18 of the two species of plants are then
immediately abutted and a thin cord is wrapped or tied about the
abutted roots in order to insure good contact between them and
maintain them in abutment. The excisions on each of the seedlings
12 and 14 should be complementary in order to maximize contact
between the cut surfaces 16 and 18. The joined seedlings 10 are
planted and nurtured to adult plants, at which time either seeds
from the plants are harvested for growth of a T-2 generation from
which plants having desired traits are selected; or the T-1 adult
plants are directly selected for desired traits. The former is the
particularly preferred procedure in this invention.
In the embodiment disclosed in FIG. 1, the seedlings 14 of the
acceptor species are exposed to electrophoretic conditions through
the existence of a difference between the natural membrane
potentials known to exist about both plant and animal cells,
Jaffe, Nature, 256: 600-602 (1977). Although natural membrane
potentials are known to be of low magnitudes, generally on the
order of 1 to 100 millivolts, the adjacent disposition of cells of
different species will result in a mutual electrophoretic process.
Because each plant species has its own distinctly characteristic
metabolic cycle and timing of activity, the biochemical cycles in
plants 12 of one species will likely be at a phase different from
that of plants 14 of the second species. Consequently, since at
one growth stage the mutual potentials may be complementary and at
another stage of development they may be opposed, this can provide
a potential gradient quite different from that which the cells of
the plants 12 of the first species would experience under normal
conditions of development.
Because the plant radicle or root tip is responsible for the
production of vitamins and other important enzymes used in the
development of germinal plants, the plant 14 having the root tip
excised will be acceptor plant, while the plant 12 having the root
tip retained will be the donor plant.
Applicant has measured the current density in the region where the
cut tissues contact, when abutted as disclosed in FIG. 1. For
example, when four day old seedlings from different species such
as corn and soybean are paired, the current density reaches a
maximum value of about 0.7 microamperes per square centimeter at
about 40 minutes after initial abutment, with a very gradual
decline over the next 10 hours. In contrast, when seedlings of the
same species are paired in a similar fashion, such as
soybeam-soybean pairings, the current density is only around 0.01
microamperes per square centimeter, again showing a very gradual
decline with time. Typically, even at this low electric potential
difference between the seedlings of disparate species, new traits
appear in the acceptor plants at about a 5% mutuation level and
are often in the nature of phenotypical alterations such as plant
shape, size and foliage color. The T-1 generation is then selfed
to yield a T2 generation, and the altered phenotypes exhibited by
the mutated members of the new generations do not segregate out in
succeeding generations.
With reference now to FIG. 2, a second preferred embodiment of the
invention is thereshown in which the natural membrane potential
difference between seedlings of two different species is augmented
or reversed, as desired, by the application of an ionophoretic
current across the joined seedlings. More particularly, the root
tips of seedlings 12 and 14 of two different species are excised,
and the cut portions of the seedlings abutted together. A pair of
electrodes 22 are then afixed to the seedlings 12 and 14 generally
opposite the abutted root portions, for example, in the shoots or
cotyledons. An electrical potential such as provided by a constant
direct electrical current is then applied through the electrodes
22 across the pair of joined seedlings 20. The voltage applied to
the seedlings will generally range between 1 to 45 volts, and
preferably on the order of 1.5 to 22.5 volts, for times of about 5
minutes to 24 hours, and preferably about 5 minutes to about 3
hours. This yields a current density across the region where the
cut seedlings abut one another in a range of about 10 to 100
microamperes per square centimeter. Preferably, the direction of
current applied is chosen to augment the difference in membrane
potential of the cells between the different species of plants.
Once subjected to such a potential, the seedling pairs 20 are then
separated into individual seedlings 12 and 14, which are
separately nurtured to adult plants. The selfed T2 generation from
the treated T1 generation plants are then selected for desired
traits.
Preferably, the electrodes 22 are constructed from iron, since
iron electrodes can be inserted into the seedlings without causing
detrimental effects to the seedlings. Other electrodes which are
not deleterious to plants can also be used, and stainless steel
electrodes are particularly preferred for this purpose.
The donor material employed in the present invention need not be a
whole plant or seedling. Instead, as shown in FIG. 3, tissues of a
donor species of plant can be macerated, such as by blending in
water, in order to produce an aqueous donor liquor. The aqueous
liquor is collected and added to a support medium such as agar or
gelatin, to produce a treated medium 26. The treated medium 26 is
disposed in a test tube or vial 28, or other convenient container,
and the root tip of the seedlings 14 of the second species of
plant are placed in contact with or immersed in the medium 26.
Most preferably, the radicle of the acceptor plant seedling 14 is
placed in the medium. Once electrode is contacted with the shoot
of the seedling, while another electrode is disposed in contact
with the support medium 26. An electrical potential is then
applied across the seedling 14 and medium 26, of the type, time
and intensity described in the preceding embodiment. Following the
application of this electrical potential, the seedlings 14 are
removed from the treated medium 26 and grown to adult plants,
which are then either selected for desired traits or are selfed in
order to determine which traits in a T2 generation are inheritable
and stable.
The use of agar or gelatin as a medium 26 for suspending the
aqueous liquor is desirable, but not essential to use of an
aqueous liquor of the donor plant cells. In the embodiment shown
in FIG. 3, the medium can be replaced by the aqueous liquor
itself. Moreover, the aqueous liquor can itself be subjected to an
electrical potential prior to its contact with the acceptor
seedling 14, as shown in FIGS. 4 through 6. As above, the tissue
of the donor species of plant is macerated in distilled water, and
the resulting liquor 30 collected. The aqueous liquor 30 is then
deposited in a petri dish 32, and a positive electrode 34 and a
negative electrode 36 are placed in the aqueous liquid 30. An
electrical potential 38 is then applied to the aqueous liquor 30
across the positive electrode 34 and the negative electrode 36.
While the electrodes can be constructed from silver, it is
preferred that the electrodes are constructed from platinum in
order to reduce oxidation of the electrodes, and minimize the
effect of the electrode material upon the aqueous liquor 30.
Generally a potential of about 5 to 20 volts is applied for a time
of about 10 to 30 minutes. Consitutents of the aqueous liquor will
migrate towards or away from one or the other of the electrodes 34
and 36, depending upon the charge possessed by the various tissue
constituents. As shown in FIG. 5, the portion of the aqueous
liquor 30 which is located about the anode or positive electrode
34 (the anode solution) is removed from the remainder of the
aqueous liquor 30 by withdrawal into a hypodermic syringe 40. The
portion of the liquor 30 surrounding the negative electrode or
cathode 36 (the cathode solution) is removed by drawing into a
syringe 42.
The syringes 40 and 42 containing the anode and cathode solutions
are then inserted into opposite ends of seedlings 14 of the
acceptor species, as shown in FIG. 6, and pressure is applied to
the syringes 40 and 42 to inject a portion of the anode and
cathode solutions to the tissue of the seedlings. For example, the
anode solution contained in the syringe 40 can be inserted into
the shoot 44 of the seedling 14, while the cathode solution
contained in the syringe 42 can be injected into the root of the
seedling 14, preferably into the radicle 46. The positive
electrode 34 and negative electrode 36 are then connected to the
syringes 42 and 40, respectively (opposite to the electrodes from
which the syringes collected a portion of the aqueous liquor), the
syringes preferably having metal tips to facilitate electrical
contact with the seedling 14. A potential difference of about 1 to
50 volts and preferably of about 1.5 to 22.5 volts is applied to
the seedling through the syringes for a time of about 5 minutes to
24 hours, and preferably for about 5 minutes to about 3 hours.
Subsequent to the application of the potential difference, the
needles are removed from the seedling 14, and the seedling 14
grown to an adult plant. A plurality of seedlings are selected for
the desired traits in either the T1 or T2 generation, as described
earlier.
In another preferred embodiment of the present invention, only one
of the electrode solutions needs to be applied to the acceptor
species of plant in order to obtain the high proportion of
mutations encountered in the present invention. With particular
reference to FIG. 7, either of the anode or cathode solutions
collected by the syringes 40 and 42 can be applied to a porous
medium, such as a filter paper 48. A seedling 14 of the acceptor
species of plant is positioned on the filter paper 48 with both
its radical 46 and its shoot 44 in contact with the filter paper
48 containing the donor electrode solution. The donor-containing
filter paper 48 is placed in contact with a first electrode 50
while a second electrode 52 of opposite polarity is inserted into
the shoot 44 of the seedling 14. As above, the polarity of the
electrode 50 in contact with the filter paper 48 is opposite to
the sign of the electrode 40 or 42 from which the anode or cathode
solution was collected. Because at least some of the constituents
of the anode or cathode solution will be of the type to migrate
towards the electrode opposite in sign so that of the electrode 50
in contact with the filter paper, these constituents will tend to
migrate towards the second electrode 52 upon the application of
the potential difference across the electrodes 50 and 52, and
thereby across the seedling 14. The length of time and type and
strength of potential difference applied across the seedling 14
are as disclosed above. Subsequent to the application of the
potential difference, the electrodes 50 and 52 are removed from
the seedling, and the seedling 14 grown to either the T-1 or T-2
generation, and selected for any desired traits. The electrodes 50
and 52 are preferably constructed of iron or stainless steel,
because of their minimal effects on biological systems.
It should be evident that the embodiments disclosed in FIGS. 3 and
7 are readily adaptable to use in exposing a single cell or
isolated protoplast cell of an acceptor species to the aqueous
liquor or cathode or anode solutions from the donor species. More
particularly, in FIG. 9 there is disclosed another preferred
embodiment of the present invention in which a pair of
non-reactive electrodes 70 and 72 (preferably platinum electrodes)
are used to place an electrical potential across a single plant
cell or isolated protoplast 74. The cell 74 is carried on the end
of a glass tube 76, the tube 76 being filled with water 78 or
another conductive liquid so as to permit manipulation of the cell
74 within the tube 76. The use of a water-filled tube to carry a
single plant cell is, of course, a known micromanipulative
technique. One of the electrodes, for example, the cathode 72, is
electrically connected through the tube 76 and disposed in contact
with the liquid 78 in the tube 76. The other of the electrodes,
for example, the anode, is electrically connected through the wall
of another glass tube 80 and disposed in contact with a donor
medium 82 contained in the tube 80. The donor medium 82 is the
same as the media prepared in accordance with the preceeding
embodiment of the invention. Pressure is applied to the medium 82
to express a small droplet 84 of the medium 82 out of the end of
the glass tube 80. The tubes 76 and 80 are mounted to a
micromanipulator (not shown), which aligns the tubes 76 nd 80 and
permits the droplet 84 to be brought into contact with the plant
cell 74. Alignment and contact can be visually monitored through a
microscope 86. The plant cell 74 is then subjected to
electrophoretic conditions by the application of a DC voltage
across the electrodes 70 and 72. The applied voltage should be
sufficient to produce a current density in the range of 1.0 to 100
microamps per square centimeter, for a time of about minutes to
three hours.
A final preferred embodiment of the general method of present
invention is shown in FIG. 8 in which a seed 62 of an acceptor
species of plants id disposed between two pieces of porous
material or filter paper 54 and 56. Cathode and anode solutions of
a tissue macerate of a donor species are prepared as described
above. The filter papers 54 and 56 are placed on electrodes 58 and
60, and infused with the anode or cathode solution collected from
the electrode 34 or 36 of potential opposite to the electrodes 58
and 60. One of the filter papers 54 or 56 is placed in contact
with the hilum or embryo end of the seed 62. The electrodes 58 and
60 can be constructed of various materials, preferably stainless
steel or other iron material. It is preferred that the electrodes
do not contact the seed 62 directly. An electric potential is the
applied to the electrodes 58 and 60, and thus applied across the
seed 62. The potential can be applied to the dry seed 62, or the
seed can be allowed to be partially or completely imbibed with
water or the anode and cathode solutions, before the potential
difference is applied. A constant direct current of 20 to 90 volts
is applied to the dry seeds, or a potential of 1 to 40 volts is
applied to the partially imbibed seeds, for about 5 minutes to 1
hour. After such treatment, the seed may be returned to the
quiescent state and stored until it is convenient to plant them.
Alternatively, the seeds may be germinated immediately, sprouted
and grown to adult plants. Adults in the T2 generation, selfed
from the T1 plants, are selected for desired traits.
The methods of the present invention are further illustrated by
several following examples. Some of the examples have been
followed through the T5 generation in extensive agricultural
testing. In general, it has been found in the invention that the
induced mutations recognizably segregate in the T2 or subsequent
generations, so that selections for further crossings or further
development can accordingly be made in the T2 generation. For the
most careful screening of the types of mutations, it has been fund
advantageous to examine plant row tests in the T2 generations,
that is, to use seeds from the individual treated plants of the T1
generation for inbred or selfed plant row replications, in the T2
generation testing. This allows a more efficient screening and
categorization of the induced mutations from the T1 generation
since traits or characteristics which are not reproduced in a
selfed or inbred generation are neither stable nor of particular
commercial value.
The high percentage of mutants obtained in the method of the
present invention allows a relatively small number of seeds or
seedlings to be treated in the T1 generation (which is also
referred to as the transduced series), on the order of 15 to 20
seedlings of each transduction polarity being examined for
differences in growth and for phenotypic variations, along with a
control group of untreated seedlings or seeds of equal number.
Thus, about one-third of the plants field tested at the time of
the T1 generation are control plants. After the T1 generation,
each of the treated lines and controls are grown in three
replicated rows of 40 to 50 seeds each within statistically
randomized test plots. Unless otherwise indicated, the Latin
Square method of randomization was employed. Subsequent to the T2
generation the lines are selected and expanded according to the
apparent importance of the new characteristics of the mutant
plants.
The acreage necessary to adequately insure that the new
characteristics are stabilized in the particular treated lines
will vary according to the percentage of mutants obtained in the
T1 generation and the number of lines that appear desirable to
investigate. For example, in 1984, applicant produced T1
transduced series of acceptor species including corn, tomato, soy
beans and navy beans. Less than a one acre test plot was required
for 124 transduction series and controls. These particular tests
were made in lower Michigan. By 1985, the subsequent T3 generation
testing involved an area of 10 acres, while the 1986 T5 generation
required over 70 acres of primary growth, in addition to
replicated tests at several locations and in several states. The
T2 and T4 generations of these transduced series were seed
expansion grow-outs in Hawaii, in order to shorten the time
necessary to achieve the T5 generation.
The following examples serve to further illustrate the present
invention:
EXAMPLE ONE
Longitudinal sections from soy bean (Glycine max) seedling roots
(the donor species) were excised in a plurality of seedlings, and
longitudinal sections including the root tip were excised from a
plurality of bush bean (Phaseolus vulgaris) seedlings (the
acceptor species). Each seedling has a radicle in the range of 1
to 6 centimeters in length, and the excised portions were of
complementary shape, such as to expose the procambium, protophloem
and protoxylem cells of each root tip. The cut portions of pairs
of seedlings of the different species were abutted and bound with
thread, as shown and described in conjunction with FIG. 1. The
pairs of joined seedlings were grown to adult plants.
One in twenty bush bean seedlings so treated resulted in an adult
plant that was shorter than the control plants and which has more
compact foliage than the control plants, characteristics which are
of commercial importance in the harvesting of bush beans. Tall
plants tend to lodge and intertwine, and are thus less efficiently
harvested. These plants also had leaves of a deeper green color
than the control plants, the fruit of these plants and these
plants exhibited greater drought resistance than the control
plants. Yields under field condition, however, were found to abe
about the same as those of the control plants. The seeds of these
plants were observed to be intermediate in shape between the soy
bean and bush bean progenator seeds.
These new characteristics were stable; they were observed without
change through seven inbred or selfed generations with no
reversion back to the height, bushiness, color, sweetness, and
drought resistance of the original and control bush bean plants.
Six generations of the mutated plants, along with an equal number
of controls, were grown under field test conditions as described
earlier. The maintenance of these characteristics for seven
generations demonstrates that the changes were inheritable. The
fact that the inbred, transduced plants do not segregate or
revert, that is, return to the characteristics of the control
plants, demonstrates that the method can provide new varieties of
plants which breed true. As will be subsequently discussed, this
non-segregating, stable nature of the growth alterations suggests
a non-Mendelian or cytoplasmic type of inheritance.
EXAMPLE TWO
Longitudinal sections from a plurality of bush bean seedlings
(Phaseolus vulgaris) roots, the donor species, and longitudinal
sections including the root tips from soy bean (Glycine max)
seedlings, the acceptor species, were excised to expose procambim,
protophlem and protoxylum cells on each seedling. Each seedling
was germinated and possessed radicles in the range of 1 to 6
centimeters in length. The excised portions of pairs of seedlings
of different species were cut in complementary shapes, and the
exposed cut portions of the seedlings were joined together to form
pairs of joined seedlings in the fashion shown in and described in
conjunction with FIG. 1. Each pair of joined seedlings contained a
bush bean seedling and a soy bean seedling. The pairs of seedlings
were then grown to adult soy bean plants, and one in ten of the
soy bean plants so grown exhibited seeds that were intermediate in
shape and color between the seeds of the bush bean and the soy
bean progenators. The leaves of the one in ten altered soy bean
plants were less lobed in shape than the leaves of the control
plants, the stem node lengths were reduced as compared to those of
the soy bean control plants, and the number of stem nodes was
increased as compared to the controls as well. This resulted in a
line of altered soy bean plants which had more compact foliage
than the control plants and was thus more resistant to lodging
under field conditions. These changed characteristics were
maintained in inbred or selfed plants grown through four
generations. Three of these generations were grown along with an
equal number of controls under field conditions.
This example is, of course, the reciprocal or reverse of the
transduction which occured in Example 1, that is, the donor and
acceptor species are reversed. Significantly, the percentage of
altered or mutated plants obtained is of the same order of
magnitude in each example, demonstrating that the method allows
modifications to be made to plants in two directions. Typically,
attempts to induct positive and viable mutations in plants by
conventional methods such as by chemical or ionizing radiation
treatments yields an expected frequency of useful, viable
mutations or phenotypic alterations of one in five hundred
thousand test plants (a frequency equal to 0.000002). Examples 1
and 2 demonstrate that the method of the invention can produce
plants having new, inheritable characteristics at a rate of 25,000
times that expected under conditions of conventional chemical or
radiation treatment. This increase in the rate of mutation is
highly significant and commercially valuable in terms of time,
space, and the volume of plants needed to be treated or exposed in
order to produce positive mutations.
EXAMPLE THREE
Tissue from the immature fruit of tomato (Lycopersicon esculentum)
was macerated in distilled water and the resulting aqueous liquor
placed in a perti dish. A pair of spaced silver electrodes were
inserted in the macerate liquor and a constant direct current
electrical potential of 9 volts was applied for 20 minutes. A
portion of the liquor surrounding each electrode was drawn into a
hypodermic syringe having a conductive needle tip. The conductive
syringe tips were inserted into the root and shoot of a plurality
of soy bean (Glycine max) seedlings in the fashion shown in and
described in conjunction with FIG. 5, 6 and 6, above. A negative
electrode was then connected to the syringe containing the
solution which has surrounded the positive electrode in the petri
dish, while a positive electrode was connected to the syringe
containing the other electrode solution. A constant DC electrical
potential of 22.5 volts was then applied for five minutes, so that
a current of approximately 100 microamps was passed through the
seedlings.
Two series of 20 seedlings were treated and grown along with 20
non-treated controls. In one treated series, the electrode
solution from the positive electrode in the petri dish was applied
to the seedling roots. In the other series, the electrode solution
from the negative electrode in the petri dish was applied to the
roots of the seedlings. After such treatments, all of the
seedlings were grown under field test conditions as described
above, and the results obtained are given in Table I below. The
asterisk indicates data which is statistically significant at
about a 95% confidence level (P less than 0.05). The observed
increases in pod and seed yields continued in two subsequent
generations of selfed or inbred plants, grown under field test
growth conditions.
TABLE I
(N = 20 plants per series)
ELECTRODE SOLUTION
PODS PER PLANT
AVERAGE SEED YIELD
APPLIED TO ROOT
AVERAGE
S.D.
(GRAMS PER PLANT)
ANODE (+) 59.1* 43.6
16.76
CATHODE (-) 49.6* 36.7
14.06
CONTROLS 32.5 13.7
9.50
EXAMPLE FOUR
A portion of root tissue from Eastern Marsh Cabbage Plant
(Symplocarpus foetidus) was excised in early Spring (mid-March),
macerated in distilled water and admixed with a sufficient
quantity of agar to create a donor macerate of moderate viscosity.
A portion of this donor macerate was placed in a test tube. The
radicles of a plurality of tomato seedling (Lycopersicon
esculentum) were immersed in the donor medium. One electrode was
inserted into each of the seedlings, while another was positioned
in contact with the donor medium. A direct current 9 volt
potential difference was applied across the electrodes, and thus
across the seedlings and macerate, for five minutes.
Tomato is well known to be one of the agronomic crops which can be
commercially grown both under greenhouse and field conditions,
while it has been noted that the Marsh Cabbage possesses a high
metabolic output in its early stages of growth, R. M. Knutson,
Science 186: 746-747 (1974). In view of the hypothetical model set
forth in the discussion following the examples herein, and in
light of the fact that certain characteristics of the transduced
plants in Examples 1 and 2 were intermediate the characteristics
of the donor and acceptor species, it was thought there was a
significant chance that the high metabolic output of Marsh Cabbage
could be imparted to tomato seedlings to increase their fruit
yields, and thereby increase the commercial value of the crop.
A number of tomato seedlings so treated were grown in a
greenhouse, and the number of plants resulting from treatment, and
the number of fruit borne by those plants at the time of fruit
ripening, are shown in Table II. Both the positive and negative
electrode orientation data were combined in the data reported in
Table II, since in this case there were no apparent polarity
differences. Again, the asterisk indicates data which is
significant at a 95% confidence level (P less than 0.05).
TABLE II
FRUIT/PLANT
DONOR AVERAGE AND s.d. N-PLANTS
Macerate
*4.33 (2.64) 15
Controls
2.43 (2.42) 21
This same donor/host transduction was repeated for the purpose of
examining yield levels under field conditions. Using three
different varieties, a total of 24 test series were prepared with
30 transduced seedlings in each series (15 per electrode polarity)
plus 15 control, non-transduced plants. Exposure was again
conducted with the apparatus shown in, and the method described in
conjunction with, FIG. 3, at a direct current potential of 9 volts
and an exposure time of five minutes. All plants were handles and
reared under similar conditions of field environment. Yields from
individual plants were recorded at the time of optimum fruit
harvest (approximately two-thirds mature fruit per plant).
Of the 24 test series, 7 of them, or 29.2%, disclosed a
statistically significant yield advantage (based on mean weight of
fruit per plant) over the control or non-transduced groups, at a
confidence level of 95% (P less than 0.05). Within the groups
showing yield increases, there were also concomitant,
statistically significant increases in growth rates and in plant
size. The yield data possessing this significance ranged from +35%
to +70% fruit weight increases over the controls. An experienced
plant geneticist and breeder observing the transduced series
selected one with a +50% (P less than 0.05) yield increase as
having improved phenotypic characteristics for traits desirable
for commercial harvesting, specifically, upright plants having
good clustering of fruit.
Individual plants were selected and grown in T2 generation field
replications as plant rows (30 plants per row) from this
particular exceptional plant series as well as from several of the
T1 generation series, including some that showed no yield
advantages. Yields were again recorded in the T2 generation. These
plants row data disclosed that those plants showing growth and
yield advantages in the T1 generation also gave high growth rates
and yields in the T2 generation, whereas those showing no growth
or yield advantages in the T1 generation gave no growth or yield
increases in the T2 generation. From the T1 generation exceptional
progenitor plant, a total of 10 plant rows gave statistically
significant yield advantages ranging from +40.7% to +55.6%,
compared with the T2 generation control plant yields. T3 inbred
generations of these high yielding plants are currently being
compared in several large scale field tests (approximately five
acres at four different locations) with two high yield commercial
varieties, as well as with the non-transduced F3 generation
controls. In all locations the transduced line is still showing
significant growth and development advantages over the
non-transduced varieties.
This example illustrates the consistency of the induced phenotypic
effects and practical increases in the rates of fruit production
in a commercially valuable crop, when the method of the present
invention is practiced.
EXAMPLE FIVE
The Symplocarpus foetidus root tissue used as donor material in
Example Four was prepared in the early spring (mid-March) growth
period, when the metabolic activity in the root was at a high
rate. Donor tissue prepared from different tissue regions of the
donor plant and taken at a later stage of maturity can have
significantly different effects on the growth rate in the acceptor
plant Lycopersicon esculentum. Tissues from the root, the
development spadix in the lower stem of the plant, and the leaf
foliage of the Marsh Cabbage were collected in mid-April, and
donor macerates were prepared as described in Example Four and
shown in FIG. 3. Seedlings from four different commercial and
established varieties of tomato plants were treated with these
macerates and with a direct current 9 volt potential and five
minute exposure. The tomato seedlings were grown under greenhouse
conditions and periodic growth data was obtained. Table III
presents data obtained at six weeks of growth which shows the
percentage of the total series which possessed growth
statistically significantly higher (P less than 0.05) than the
corresponding control series.
TABLE III
DONOR TESTS WITH SIGNIFICANT TISSUE GROWTH N-TEST SERIES
Root 2.8% 36
Spadix 19.4% 36
Foliage
30.0% 20
The foliage employed as a donor, with its high rate of protein
synthesis, yielded the highest percentage of tests showing
significant growth increases in the acceptor series, when compared
with the controls. It is noteworthy that the root macerate used in
the above test produced significant growth increases in only 2.8%
of the series, whereas in Example Four the root tissue obtained
about one month earlier (when at its high level of metabolic
activity) induced high growth in 29.2% of the test series.
This example serves to illustrate the importance of the selection
of tissue for the transduction donor, as well as considering its
state of maturity.
EXAMPLE SIX
Many varieties of plants in the pea and bean family (legumes) have
the ability to more efficiently utilize or fix nitrogen from the
atmosphere than other plants. This diazotrophy occurs through
bacteria which live symbiotically on the plant roots and form
outgrowths or root nodules. The results of this example suggest
that an acceptor species in the cereal family such as corn, which
does not fix nitrogen, could have mutations and growth stimulation
induced therein from a donor bean species which has these root
nodules.
A donor extract was prepared from soy bean (Glycine max) root
nodules excised from plants grown from seeds which were initially
inoculated with the bacterium Rhizobium japonicum, which is known
to produce diazatrophy in soy beans. The macerated nodule liquor
was mixed uniformly with agar as a base, and corn (Zeas mays) and
sunflower (Helianthus annuus) seedlings were both treated with
this donor extract in fashion shown in and described in
conjunction with FIG. 3.
EXAMPLE SIX (A)
Both corn and sunflower seedlings were placed in the base medium
and exposed to a potential giving an initial current of about 30
microamps through the seedlings. After exposure the seedlings
(along with equal numbers of controls) were planted in a field
test plot, with no fertilizer added. Growth and development
studies were conducted on three separate test series of corn and
two separate test series of sunflower seedlings. The growth and
development enhancement produced by the root nodule extract
treatment was consistently observed in all five test series.
Examples of growth and development data are presented in Table IV
for a field test series of corn and in Table V for a field test
series of sunflower plants. The corn seedlings were exposed to the
current for one hour, with the cathode inserted into the donor
medium; the data of Table IV were obtained from twelve plants in
each series. The sunflower seedlings were exposed to the current
for 30 minutes, with the cathode inserted into the donor medium;
the data of Table V were obtained from twenty plants in each
series. The differences in growth shown in the last column of each
table were significant at the 99% confidence level (P less than
0.01).
TABLE IV
DAYS AFTER ROOT NODULE EXTRACT
CONTROLS GROWTH
PLANTING AVE. S.E. AVE. S.D. DIFF.
7 9.25 cm
2.14 cm
5.09 cm
1.64 cm
+81.7%
19 47.00 6.41 31.45
8.89
+49.4%
31 83.58 9.99 64.64
16.83
+29.3%
46 104.92 13.14 80.45
19.44
+30.4%
62 109.00 25.04 74.64
37.37
+46.0%
After 73 days of field growth, the root nodule group disclosed an
84% near development and the control group only a 16% ear
development. After 90 days field growth, the average ear weight of
the root module series was 55.0 g and the average ear weight of
the control series 28.7 g. The kernals on the treated series were
also more fully developed than were those of the controls.
TABLE V
DAYS AFTER ROOT NODULE EXTRACT
CONTROLS GROWTH
PLANTING AVE. S.D. AVE. S.D. DIFF.
10 8.00 cm
1.56 cm
6.00 cm
1.81 cm
+33.3%
22 20.30 3.15 16.00
3.89
+26.9%
37 67.00 10.87 56.25
11.18
+19.1%
53 103.10 16.41 86.90
18.17
+18.6%
At maturity the mean seed pod weight (before seed removal) of the
nodule-treated group was 21.7% higher than the mean seed pod
weight of the control group.
In these field test series, the polarity conditions were limited
to the donor medium electrode being the cathode. The reason for
examining only the one polarity condition was the fact that
preliminary studies with the soybean root-nodule extract disclosed
a greater growth response with the donor medium media electrode
being negative than with the medium electrode being positive.
This example shows the induction of more efficient growth,
development and yield from the root-nodule extracts, as compared
to the control plants.
EXAMPLE SIX (B)
Corn seedlings were placed in the donor medium and exposed to a
direct current 15 volt potential giving an initial current in the
range of 30 microamps through the seedlings. After 10 minute
exposures, groups of 15 test seedlings along with equal number of
controls were planted in a field test plot, no fertilizer added,
and growth data taken periodically during the growth cycle. The
test was conducted with five pure and commercially available
inbred varieties, Mo17-Ht, A634-Ht, A632-Ht, B73-Ht and W117-Ht,
and thus provided range of different lines of stable but
homozygous test material. The use of different inbred lines also
provided a germ plasm for subsequent hybrid crossing studies.
A total of 18 test series were prepared and examined under field
conditions in accord with this protocol. The observed development
alterations in the T1 generation were primarily in the rates of
maturity or tassel development, in growth rates and in changes in
root structure and morphology. The roots in several of the treated
series disclosed a much more branching or dendritic patterning,
with thickening at the terminus of the root. The roots of the
control plants had less branching with no thickening at their
termini. This formation of inchoate nodules and alterations in the
root morphology of the corn plant is indicative of the initial
stages of diazotrophy induction in this cereal plant. Plants from
those groups disclosing significant increases in the rate of
tasseling or growth were then selected on an individual plant
basis for T2 generation self pollination. These T2 general plants
were then used in T3, T4 and T5 generations, for both inbred and
hybrid crosses.
The advantage of the use of genetically pure, homozygous inbred
varieties or lines as the acceptor materials is that mutations in
corn can be keyed to alterations in particular chromosomes from
known listings. Specifically in the case of corn, new genotypic
and phenotypic expressions can be compared with those listed in
The Mutants of Maize, N. G. Neuffer, et al Crop Science Society of
America (Madison, Wisc.), 1968; and Maize for Biological Research,
W. F. Sheridan, Ed. Plant Molecular Biology Association,
(University Press, N. Dak.), 1982. It is well known to those
skilled in the art that if a particular characteristic appears in
a subsequent generation of a plant line where this characteristic
was not previously present, a point mutation has occurred on a
particular chromosome. Indeed, these point mutations are cataloged
in this fashion.
The treatment of corn seedlings by the present invention with the
soybean root module donor material produced a number of changes in
characteristics in the T2 generation which are known to be
associated with particular point mutations. In particular, a
number of these mutations are known to be located on chromosome
which occurred in the T2 generation plants obtained from some of
the treated series. A summary of the frequency of mutations found
in two of the inbred treated series (derived from the A632-Ht
acceptor) are listed below in Table VI. There were no mutations or
phenotypic alterations (a zero percent level) observed in several
thousand control or non-transduced plants from this same inbred
line.
TABLE VI
CHROMOSOME-3 POINT MUTUATIONS
(T2 GENERATION FROM TRANDUCED INBRED A632-Ht)
MUTATION SERIES M33-1-18
SERIES M33-1-7
Dwarf 7 (20%) 0
Short 7 (20%) 0
Dwarf-Crinkly Leaf
5 (14%) 13 (30%)
rinkly Leaf 0 8 (19%)
Short-Romosa 0 8 (19%)
Dwarf-Crinkly-
0 1 (2%)
Romosa
Normal 16 (46%) 13 (30%)
TOTAL PLANTS 35 43
The probability of any one of these mutations occuring in one
plant by change alone is about 1 in 500,000 whereas in Table VI
there are shown several cases in which a number of plants
expressed two mutations and in one case, a single plant expressed
three mutuations. Now, from the laws of strict probability, the
odds that these percentages occurred by random chance are, in the
case of two mutuations on the same plant, one in 2.5.times.10@11
and, in the case of three mutuations on the same plant, one in
1.25.times.10@17. In addition to point mutuations, other
transduced series were observed to express large increases in
point mutuations which are known to involve several gene alleles.
Examples of these multiple allele mutuations are listed below in
Table VII.
TABLE VII
MUTATION TRANSDUCED SERIES VAR-
PLANTS ALBINO LUTEUS IEGATED TOTAL
MED. 27 26% 27% 0 144
(A632-Ht)
MED. 25 0 0 10.7% 28
(A632-Ht)
CONTROLS 0.024% 0.037% 0% 8179
(A632-Ht)
The data of Table VII show a mutuation increase for both albino
and luteus of about a thousand times the level observed in the
control population. Many of these mutations are not of commercial
interest. For example, albino plants do not produce chlorophyll
and expire before maturity. However, there were other mutations
which have importance in plant breeding. The dwarf plants listed
in Table VI are an example of a useful mutation. These plants are
about one half the height of the control plants, but the ear size
and production were comparable to those of the controls. This
normal ear size on the mutuant dwarf plants is an important and
commercially beneficial distinction from dwarf corn plants derived
from conventional breeding programs, the difference being that the
ears on the conventionally bred dwarf plants are small when
compared with the normal hybrid ears, and have large areas on the
ears which do not develop kernels at all.
Field studies of dwarf plants obtained from corn seedlings treated
in accordance with the method of the present invention establish
the existence of a number of commercially important
characteristics. The following has been shown to be true from five
generations of field trials:
The inbred, dwarf mutants have held their recessive
characteristics through the T5 generation and exhibit a 50%-60%
reduction in plant height, when compared with untreated parent
inbred control corn plants, yet produce full ears of normal size,
as compared to the controls.
Using this same method and the same soybean root-nodule macerate
as the donor material, the dwarf traits have been produced in
treated series from four of the five original inbred varieties.
When T5 generation dwarf plants originating from two different
treated inbred lines are crossed in a normal manner to produce a
hybrid, the dwarf characteristics are transferred to the hybrid.
The resulting hybrid is uniformly about 40% of the height of the
hybrid resulting from a cross between two untreated, inbred parent
lines.
The ear size and kernel formation in the dwarf hybrids are about
the same as in the untreated hybrid controls. The commercial
significance of this is that a smaller plant size in the dwarf
hybrid allows a higher plant density under field planting
conditions, which in turn results in a higher yield per acre.
Lastly, the ears on the dwarf plants are located much lower on the
plant than on the normal or control hybrids, and thus are more
efficiently harvested than those on taller control plants.
Additionally, a male sterile, cytoplasmic mutation (Cms) was
observed in 100% of the plants in one of the transduced, Mo17-Ht
inbred lines. This mutation is commercially important in the
development of inbred lines which do not require the laborious
task of de-tasseling in the normal production of hybrids.
In the T3 generation, a number of plants selected for phenotypic
growth and yield advantages were used for hybrid crossing studies.
In general, the early development and high yield traits present in
the T3 generation plants were transmitted into the hybrids when
the treated progeny were expressed through the female line of the
hybrid. An example of this is a soybean root-nodule donor series
expressing the mutuation "prolific", which relates to the
percentage of plants with multiple ears. A normal hybrid line has
about a 10% level of prolific plants. In hybrid crosses, using
female parents from T2 generation inbreds, a direct correlation
was observed between the percentage of plants with prolific
mutations and the resulting yields. The yields from three field
replications were compared with a good producing commercial
hybrid. The yield from one of these high producing treated lines
is compared with the control hybrid in Table VIII below.
TABLE VIII
PRO- YIELDS
HYBRID SERIES LIFIC (g/plant) YIELD INCREASE
Control (HL2454)
8% 200.09 --
Female Transduced
47% 257.87 +28.9% (P < 0.05)
This example illustrates the number and type of mutations which
can be induced by the methods of the present invention. Many of
the mutuations have utility in the production of new varieties and
in the hybridization of plants. The useful mutant characteristics
are selected from the test populations by conventional segregation
testing methods commonly employed by plant breeders. The useful
mutations are also expressed when employed in hybrid crosses.
EXAMPLE SEVEN
As noted in Example Six, the Eastern Marsh Cabbage (Symplocarpus
foetidus) has a high metabolic output during early spring growth,
the result of which is development of the plant during a period of
temperatures too low for growth to proceed in most plant species.
This metabolic response can be imparted to corn (Zea mays) by the
method of the present invention, when a donor extract from Marsh
Cabbage is applied to the corn seedlings. Potential benefits of
such a characteristic might be expressed as higher yields, faster
development rates or other useful mutations. A new variety with
some or all of these attributes could be grown in regions of the
world where the growing season is conventionally believed to be
too brief for corn development.
EXAMPLE SEVEN(A)
A donor medium was prepared from the macerated roots of the Easter
Marsh Cabbage, and corn seeds were exposed to a direct current
during initial inbibition with the medium with the apparatus shown
in, and by the method described in conjunction with, FIG. 8. After
treatment, the extract-exposed and control series were examined
under field growth conditions. Table IX discloses growth data
taken just before mid-maturity (36 days after exposure). Each
series contained 16 plants. Only the series having a positive base
plate polarity during exposure of the seeds exhibited a
statistically significant increase (P less than 0.05) in growth,
as compared to the controls.
TABLE IX
BASE PLATE PLANT GROWTH PERCENT POLARITY AVE. S.D. CHANGE
(-) 0.878 0.284 +9.5%
(+) 1.003 0.234 +25.1%
Controls 0.802 0.134 --
The polarity differences shown here are consistent with those
mentioned in Example Six(A). With the base of the apparatus being
the positive electrode, the embryo or radicle end of the seed was
disposed upwardly, in contact with the cathode. This arrangement
is the one which exhibited a statistically significant increase in
plant growth. Cathode-radicle exposure was also the optimum
situation for the plant series reported in Table IV and V. This
demonstrates the consistency of the electrode orientation in the
method of the invention.
A detailed field examination of the plants listed in Table IX
disclosed five unique plants out of each group of 16 treated
series. Each of these five plants had definite growth enhancement,
larger and greener foliage, the foliage being more pronounced than
even the other members in the same test series. The growth of
these designated "sub-groups" are listed in Table X, again at 36
days after exposure. The differences in growth between the
sub-groups and the controls were statistically significant (P less
than 0.01).
TABLE X
SUB-GROUP PLANT GROWTH GROWTH
POLARITY AVE. S.D. N-PLANTS DIFF.
(-) 1.208 m 0.039 m 5 +50.4%
(+) 1.238 0.070 5 +54.4%
Controls 0.802 0.134 16 --
Displayed in Table XI are data showing the differences between the
leaf blade width in the two sub-groups and the controls. These
data were taken at nodes 6 and 7 at 106 days of maturity. The
differences in leaf width between the treated and control series
are statistically significant (P less than 0.01).
TABLE XI
LEAF
SUB-GROUP MAX. WIDTH WIDTH
POLARITY AVE. S.D. N-LEAVES DIFF
(-) 9.29 cm 0.91 cm 16 +15.6%
(+) 9.44 0.87 16 +17.4%
Controls 8.04 0.79 16 --
Development was also more rapid in these sub-group plants. At 82
days development, both sub-groups disclosed 100% tassel formation,
whereas in the controls only 37% possessed tassels. The positive
polarity sub-group also disclosed two developing ears, with no ear
development at all in the controls.
The final yield results for each entire series of plants is shown
in Table XII. The ear weights are somewhat lower than normal,
especially in the control series. This was due to a dry period
during early ear development, a situation which occurred
throughout the Midwest in the 1983 growing season. All three
series were, however, subjected to the same water stress
conditions. The data in Table XII show the importance of early ear
development in the two test series which occurred before the water
stress interval.
TABLE XII
BASE PLATE EAR WEIGHT WEIGHT
POLARITY AVE. S.D. DIFFERENCE
(-) 78.5 g 51.3 g +187.6%
(+) 131.4 80.2 +381.3%
Controls 27.3 24.2 --
The final ear weights from the two sub-groups of special high
vigor plants gave values of 134 g per ear for five negative base
plate polarity plants, and 230 g per ear for the five positive
base plate polarity plants. The controls averaged only 27.3 g per
ear. The differences are significant at a 99% confidence level (P
less than 0.01).
This example demonstrates the induction of a metabolic response
having a positive effect on both development and yield in corn,
when the corn seeds are treated in accordance with the method of
the present invention. It also shows the practical value of
selecting outstanding plants in a given test series.
EXAMPLE SEVEN(B)
A donor medium was prepared in mid-March from the macerated roots
of the Easter Marsh Cabbage. Corn seedlings were exposed to the
donor macerate with the apparatus shown in, and in accordance with
the method described in conjunction with, FIG. 3, and with the
test conditions described in Example Six(B). The same five inbred
lines, also as described in Example Six(B), were utilized. After
exposure the treated and control series of plants were examined
under field test conditions as outlined in the previous examples.
In the T1 generation, the plant alterations in the treated series
of plants were expressed as increased development rates, plant
size and plant shape variations. From these treated series of
plants, individual plants were selected for T2 to T3 generation
inbred and hybrid crosses. In the T2 generation, several point
mutations were observed, and their degree of expression is listed
in Table XIII, along with the associated allele and chromosome on
which the mutuation is known to occur. None of these mutuations
was found in several thousand untreated controls.
TABLE XIII
MUTATION
CHROMO- RECENT
NAME ALLELE SOME NO. EXPRESSION
Rust Resistant
Rp 10 100%
Zebra Necrotic
zn 10 10%
Purple pl 6 50-100%
Pigmy pv 6 25%
Male Sterile
msl 6 90%
Defective de16 4 25%
Endosperm
From this list there are three point mutations of utility in the
commercial production of hybrids, namely, rust resistance, pigmy
and male sterile. The pigmy plants are of quite different
phenotype (narrow leaf and other known characteristics) from the
dwarf mutants discussed in Example Six(b). However, they could be
utilized for a similar purpose, to produce smaller sized hybrids
and provide higher plant densities with higher yields. The utility
of male sterile plants was discussed in Example Six(B) as well.
In addition to the mutations listed in Table XIII, two important
phenotypic alterations were observed which continue to be
expressed into a T5 generation currently under study. One new
trait involves a line with a maturity which is 12-14 days earlier
than the untreated controls. The second is a "broad leaf"
expression with leaf widths on the treated lines over 40% greater
than those on the untreated controls. The useful nature of the
broad leaf characteristic lies in the ability of the plant to
receive and utilize more radiant energy per unit time during
photosynthetic activity. The result is plant with a more efficient
and higher biomass output.
A number of treated series from both the early and broad leaf
lines were used in hybrid crossing studies. These plants were
selected for either enhanced growth or for altered plant size.
When the female line was the treated series, a number of
statistically significant yield increases and early maturing lines
were observed in the replicated field tests.
This example and previous examples together demonstrate that
different donor materials produce significantly different
mutations and phenotypic growth responses, as may be seen by
comparing the point mutations in Example Six(B) (resulting from
soybean root nodule donor material) with those in this example,
employing Symplocarpus foetidus as the donor material. New germ
plasm is constantly of importance in commercial plant breeding
programs and Examples Six(B) and Seven(B) illustrate that although
the advantageous expression of a mutation, such as male sterile,
may produce similar results, the fact that different alleles are
involved in the two examples means that the characteristics in the
germ plasm would be expressed quite differently in hybird usage.
CONTROLS
In order to insure that the results obtained in these examples
resulted from the combination of subjecting the acceptor species
plants to electrophoretic conditions and to whole cells and
associated materials of a second species of plant, controls were
conducted in which the materials of the second species of plant
were replaced by distilled water or by a macerate of plants of the
same species as the first species. Additionally, seedlings of the
same species had root portions excised and joined together, as
well as being exposed to an electropotential difference only. In
all cases, no statistically significant difference was seen
between any of the plants so treated and untreated control plants.
Thus, the results obtained in the examples described in this
application necessarily resulted from the inclusion of a donor
material from a second, different species of plants.
DISCUSSION
The data obtained in the above examples leads to the inescapable
conclusion that the frequency of plant mutations can be increased
by exposing plants in their germinal phase simultaneously to
electrophoretic conditions and to the whole cells and associated
materials of a second species of plant. The fat that some of the
mutated plants obtained possess characteristics which appear to be
characteristics associated with the donor material of the second
species of plant suggests that some genetically associated cell
tissue components or macromolecular complexes from the donor
species of plant are transferred to or transduced into the intact
living cells of the acceptor species of plant, in such a manner as
to alter the genotype and/or phenotype of the acceptor, to
allowing such altered genetic and phenotypic characteristics be
transmitted to successive generations as point mutations or as
cytoplasmic transmitted traits. The subsequent discussion and
examples supporting such a theory should be taken as evidence of
the theory; however, the theory of transduction of genetic
materials is not in and of itself essential to an understanding of
or a practice of the methods of the present invention. Those
methods have been demonstrated by the preceeding examples to be
useful in producing an increased number of mutants in a plant
population, without regard to whether the instant explanation of
how such mutations occur is correct. The fact that the mutations
occur is sufficient support for the invention.
The theory as to how the present invention operates is
straightforward. It is believed that in the present invention the
application of electrophoretic conditions to the cells of an
intact organism or whole plant allows the tranduction of
genetically associated cell tissue components and macromolecular
complexes from the donor species material to the recipient plant
species. Migration of these materials would be induced by
transmembrane ionophoretic currents, either arising from the
natural difference in membrane potentials between cells of
different species, or from an externally applied current. The
theoretical feasibility of electrophoesis occurring laterally or
along, but not through, cell membranes, has been discussed by
Jaffe, Nature, 265: 600-602 (1977), and was demonstrated
experimentally within the cell membrane and wall by Woodruff and
Telfer, Nature, 286: 84-86 (1980). However, as opposed to the
present invention, this ion migration was observed and performed
by the injection of fluorescent trace-proteins through the cell
membranes of an insect ooctye, where they were observed to migrate
laterally along "intercellular bridges" or openings, but was not
transferred through the membrane barriers without breach of them.
Quite simply, the advantage of the present invention is the fact
that it is conducted with normal, intact cells of the acceptor
species, and at worst with tissue macerates of the donor species.
The need to breach or remove the cell wall encountered in all
previous techniques is avoided.
Electrophoresis can alter cell plasmalemma permability. This
permability is changed by altering the size or current of charge
carrier proteins and micropores in the plasmalemma and nuclear
envelope. And, as demonstrated by the subsequent examples, it is
also clear that the application of electrophoretic conditions
allows the ready passage of nongenetic materials through the
normal, intact cell wall. Additionally, routine commercial gel
electrophoresis techniques demonstrate that some sort of genetic
alteration is associated with the method of the present invention.
The transmission of certain enzymes, mRNA or tRNA from the cells
of the donor plant species to the cytoplasm or nucleoplasm of the
cells of the acceptor species of plant alter the rate or path of
one or more specific biosynthetic pathways in the acceptor
species, which would then alter the phenotype of the cells of the
plant. A model of such alteration is shown in FIGS. 10A and 10B,
and is described further below.
An examination of electrophoretic technology as a testing
procedure was conducted in 20 transduced corn lines and five
untreated control lines, from which the test lines were derived.
All lines were from the T4 generation of field testing. Gel
electrophoresis indicated the presence of 10 transduced lines, or
50% of the total test group, having altered gene alleles. All five
inbred controls displayed uniform, unchanged electrophoretic
patterns. A total of 8 enzymes, out of the 37 known loci in corn,
were examined and provided confirmatory evidence of polymorphism
or new gene alleles. In a second test series, 12 enzymes were
examined. The test group consisted of 42 transduced lines, 21 from
each; of two different in-bred host or control lines. The material
from the T4 generation again possessed a high percentage, about 28
percent, of transduced lines having altered alleles, with
essentially unaltered or homozygous patterns in the untreated
control samples.
Applicant has observed that induced dielectrophoretic properties
or long range dipole interactions of a donor material can
influence the spatial configuration of organelles within the
acceptor cells located within the tissue regions of tranduction.
For example, when donors are employed which have a strong,
positive dipole charge, that is, a dipole moment much higher than
that of water, or donors are employed that have been oxidized and
thus receive a net positive charge, those donors migrate from the
anode region and pass through the plasmalemma, and associated with
the cell nucleus, forming a non-uniform electric field having a
maximum intensity at the nuclear membrane. This results in an
increase in the frequency of the collection of chloroplasts and
other cell organelles in distinct proximity with the surface of
the nucleus. In normal, untreated tissue, the nuclear-organelle
clustering is observed at a low frequency of perhaps 1%-5% of
cells, while in transduced tissue, the frequency in limited
regions around the electrode contract zone is observed to be as
high as 80%-90% of the cells. Chloroplasts and other organelles
are clearly attracted to the nuclear membrane by long range dipole
interactions.
Applicant has also observed that the chloroplasts and organelles
clustering around the nucleus is not a unique property of one
specific donor material. For example, other less dipole substances
such as distilled water, when used as a donor, do not produce the
nuclear-organelle clustering. In the case of a donor which enters
the free space (apoplast) of the host tissue and has a marked
dipole moment, but is inert with respect to passing through the
plasmalemma, the influence on the spatial patterns of chloroplasts
is quite different. In such a case the chloroplast and organelle
clustering around the nucleus is not observed, but rather the
collection of the donor material in the free space of the cells
causes a mass migration of the organelles to the cell wall, the
direction depending upon the charge characteristics of the donor
material. These changes in configural associations caused by
electrophoretic conditions greatly increases the probability level
for the exchange of genetic information between the nuclear and
cytoplasmic DNA, since the organelles are disposed in proximity
with the nucleus. The cooperative, long range dipolar effects
occur inside the cell through the microdielectrophoretic
interactions between the cell organelles. The existence of such
dipole interactions has been postulated by Pohl,
Bioelectrochemistry, Plenem Press, New York (1980). By using a
ferroelectric material, specifically, barium titanate, Pohl was
able to demonstrate dipolar attraction on the outside surface of
animal cells. However, as far as the Applicant is aware, the
instant observations are the first time that
microdielectrophoresis has been observed inside living cells.
Applicant believes the following mechanism may be an appropriate
explanation for the observed migratory phenomenon. It is well
known that the plant cell wall contains polysaccharides which act
as growth and development regulators and chemical messengers. As
noted by Albershime and Darvill, Scientific American, September,
1985, page 58, these regulatory molecules are released from the
cell wall by enzymes. Different enzymes release different
oligosaccharides (small polysaccharides). In a transduction from a
new donor species, a donor enzyme complex enters the cell wall
matrix and triggers the release of a quite different array of
oligosaccharides which, after entering the cytoplasm, redirect
patterns of development and form different genotypic associations
with either the cell nucleus or the cytoplasmic organelles. As
microdielectrophoresis takes place as described above, both
nuclear and cytoplasmic interactions occur. This redirection of
growth regulators from the cell walls could not occur in the
recombinant DNA or protoplast fusion technologies, since the cell
wall is necessarily removed in the early stages of the techniques.
Further, the enzymes of one plant species may act as isoenzymes of
the second plant species and possibly alter the morphogenic
properties of the cell. Indeed, there may be enough of a potential
difference between the cells of a difference species to facilitate
the formation of intercellular cytoplasmic bridges which may allow
certain cytoplasmic extranuclear DNA or cell organelles to be
transferred from one species of plant to another. The transferred
cytoplasmic extranuclear DNA and organelle systems would also
exert some influence over the morphogenic determinative
components, thereby transforming the phenotype of the tissues.
Additionally, phygocytosis may occur and invaginate certain cell
organelles through the cell plasmalemma and into the cytoplasm.
Because the cell organelles and cytoplasmic extranuclear DNA
synthesize at least some proteins and other materials, which are
vital to cell function, the addition of cytoplasmic extranuclear
DNA and cell organelles from a different species of pant may cause
the creation of enzymes and proteins which are similar enough to
the transformed cells' natural products to be utilized by the
transformed cell but may, in the process, act as isoenzymes and
"isoproteins" which cause the plant to exhibit different
phenotypic characteristics, which may then be transmitted to
successive generations in a non-Mendelian fashion. For example, in
the technique described in conjunction with FIG. 3, the maceration
of the donor tissue in distilled water liberates proteins and
enzymes inside the cytoplasm of the donor cells, and this can
facilitate the transfer of these constituents, because such
constituents need pass only from the medium through the cell wall
and plasmalemma of the host into the cytoplasm, rather than having
to pass through at least two whole cell walls and plasmalemma, as
would be the case for non-macerated donors.
The procedures of this invention are believed to involve
transductions within the somatic tissues of the host material. The
complete expression of a new mutation or phenotypic alteration is
not usually observed until at least the T2 generation. For this
reason any explanation of what takes place in the host plant after
the application of any of the described procedures cannot be based
on the concept of a direct, abrupt uptake of donor DNA into the
host plant cells during the initial transduction process. The
establishing of a fixed genetic expression arising from a
transduction appears to be a very gradual process and is believed
to occur in a series of stages during the entire cycle of plant
development.
For the gradual incorporation of a new genotypic or phenotypic
expression into the host plant, the transductions are assumed to
be operating within specific biofeedback control systems involved
in the plant morphogenesis. To convey this proposed concept of
perturbations induced by the genetic transduction process, the
least complex of known homeostasis pathways is adopted as a model,
B. C. Goodwin, Temporal Organization in Cells, Academic Press, New
York (1963). In this simple pathway the alteration takes place at
a single active gener locus G@0, which mormally leads to the
synthesis of a cellular metabolite m@0 (or enzyme according to the
scheme shown in FIG. 10A. In this model, m@0 acts as a repressor
or co-repressor at the gene site G@0 through the feedback loop.
The main concern here is with the control of protein (enyzme)
synthesis Y@0, which regulates the final production of the
cellular metabolite.
The assumption is made that the level of the metabolite m@0 is
perturbed by the introduction into the cell of a homologous
metabolite from a different plant species by means of the
transduction process. This new metabolite m@x acts at the cellular
locus and augments the concentration of m@0 so that the new level
is at the concentration m@1 (FIG. 10B) after the transduction is
completed. The rate at which the effect of the transduction m@x is
annulled is, for a small perturbation, proportional to the
magnitude of the disturbance. From first order chemical kinetics,
the level as a function of time t after the transduction is
m@x =a(e@-kt)
where a and k are constants. A very important point here is that
m@0 and m@x must be homologous proteins and very similar in their
biosynthetic activity in both the donor and host plant systems. if
this were not the case, the control loop m@0 and repressor level
would be unaffected, or in the case of an incompatible metabolite,
the entire loop could be inactivated. This could readily explain
shy some species are effective as donors and others are not, and
why different tissue regions of the same species respond different
as donors.
The perturbation of the normal metabolite concentrations m@0 to a
new level m@x would, through the feedback control, alter the rate
of mRNA synthesis at the gene site G@0, and a new rate of
metabolite production would be established in the tissue of the
host plant. As the somatic tissues develop, the entire pattern of
gene expression during plant morphogenesis is operating at a
different level of temporal organization of nucleotides than would
be found in the non-transduced system. As this perturbed,
transduced tissue differentiates into meristem regions and
ultimately into germ plasm, the kinetics of these altered
biosynthetic pathways are transcribed as altered gene alleles,
with permanent expression being established in the DNA code.
During transcription, the mRNA would contain altered codon sites,
which in turn would lead to altered protein synthesis as the
polypeptide chains are synthesized on the ribosome surface. Thus
we have the situation of the induction of new enzymes synthesized
in the epigenetic cycle or enzymatic adaption through the
introduction of homologue precursors from another plant species
(the donor).
The perturbations of biofeedback control mechanisms within more
complex co-repressor systems could account for incomplete or
partial masking of dominant alleles in the somatic tissue. In the
situation where cytoplasmic mutations arise form the
transductions, the inherited alterations may be brought about in
quite a different manner. In this case the presence of foreign
polypeptides from the donor leads to the possibility that such
polypeptides become genetic precursors and may be subsequently
imported into chloroplasts and mitochondria, A. Cashmore et al.,
Biotechnology, 3: 803-808, (1895). The plant genome is unstable
and capable of generating variability, Science, 224: 1415, due to
changes in repeated DNA units which are more common in plants than
animals (more than 75% of all DNA sequences fifty base pairs or
longer is repetitive DNA). Repeated sequences are especially prone
to undergo loss or gain because they can promote the incorrect
pairing of chromosomes during meiosis. If there are multiple
copies of a gene, one copy may be mutated and lead to a new
function, as in the above transduction scheme, while the previous
function is maintained by the remaining members or copies of the
gene. Such copies have the characteristics of transposable
elements, B. Mc Clintock, Science, 226: 946, with the result that
some specialized cells undergo gene activation and phenotype
changes. Only DNA loss is irreversible, other DNA alterations such
as methylation, chromatin structure, protein-DNA interactions and
the like being reversible and modifiable. The mechanisms for all
embodiments of this invention are thought to be similar to the
above recited model.
Thus, under the application of an electric current across tissues
from two different species of plants, transmembrane ion migration
occurs, with specific enzymes, their precursors mRNA and tRNA, and
regulatory polysaccharides being transmitted from a donor species
into the cytoplasm of an acceptor species. Current flow across the
tissues also effects the electric charges on the cell membranes
and greatly alters membrane permeability and ion pathways through
the intrinsic proteins within the cell membrane, which control the
transfer of ions and large molecules. With in the cell,
microdielectrophoresis alters spatial configurations of the
organells, resulting in increased probabilities for the transfer
of genetic information between the organells and thereby causing
increased rates of mutation. The following examples demonstrate
the ready degree of ion migration occuring in cells and germinal
plants upon the application of electrophoretic conditions.
EXAMPLE VIII
To elucidate the mechanisms occuring at the cellular level, donors
were utilized with known ionic charge characteristics and with
both inert and biologically active properties. One type of host
tissue consisted of the chlorophyll containing stems of
Pelargonium maculatum. Stems about 5 centimeters long and 5 to 8
millimeters in diameter were subjected to two to four hours at
about 10 to 20 volt potentials and a current density of about 30
microamperes per square centimeter. The negatively charged, red
protein pigment from the Amaranth plant was applied as a donor
material in the apparatus disclosed in and according to the
technique described in conjunction with FIG. 8, with each end of
the host stem contacting a pigment-containing electrode. At the
cathode end of the test stem the red pigment migrated through the
section, leaving a zone of stained tissue extending several
millimeters into the stem. At the boundary of this zone of
migration, a microscopic examination revealed the stain collecting
of the nucleus of the parenchyma cells. At the anode end of the
test stem, the pigment was oxidized and because positively
charged. As it migrated from the anode end of the stem it gave the
host tissue a dark grey color zone extending several millimeters
into the stem. At the boundary of this zone, a microscopic
examination revealed a clustering or proximal grouping of
chloroplasts in the immediate vicinity of the cell nucleus. The
oxidized Amaranth was observed to collect on the nucleus, and
through long range dipole intractions (microdielectrophoresis)
formed a positive electrical field gradient which then attracted
the negatively charged chloroplasts to the surface of the nucleus
at the locations of maximum field strength.
Confirmation of these dielectrophoretic alterations in spatial
configurations of cell organelles was observed when using a
powdered form of carbonyl iron having a particle size of one to 10
microns, with a positive electric charge. When transduced into
geranium stems, as in the preceeding example, an electrophoretic
migration of 1 to 2 centimeters occured at the anode end of the
stem. Carbonyl iron is biochemically active and was observed to be
transduced into the cell cytoplasm where, as in the case with the
oxidized Amaranth pigment, it caused a long range dipolar
attraction of the chloroplasts in the cytoplasm. The chloroplasts
were found to be more tightly grouped around the cell nucleus than
in the case with the cell Amaranth donor. This is explained by the
fact that within the same host tissue and under the same
conditions of voltage and time, when compared with the Amaranth,
the carbonyl iron migrates over two times the distance into the
host tissue. This indicates that the carbonyl iron has a higher
ionic mobility than the Amaranth pigment.
A donor macerate of Phaseolus multiflora leaves containing
macromolocules and proteins with associated charge groups, when
electrophoretically transduced into the non-chlorophyll tissue of
Zea mays radicles using the same method as above, causes a
clustering of cellular plastids and other cell organelles (too
minute to identify microscopically) around the cell nuclei in the
anode region of the host tissue. At the cathode region the cell
nuclei had a smooth outline and the chromatin structure was
uniform. Other less ionic donor substances such as distilled
water, when transduced in a similar manner, with the host tissue
being either the geranium stem tissue or the non-chloroplast
radicle tissue of corn, did not induce the observed spatial
readjustments in the cell organelles.
Barium ferrite of particle size 1.3 microns and having a net
negative charge was then used as a donor for the purpose of
examining a biochemically inert substance which enters only the
free space (apoplast) of the host tissue. Using the geranium stem
as the acceptor and employing exposures as in the preceeding
example, the extent of the migration was far less than when using
the more biochemically active materials. The dark stained tissue
region was only two to three millimeters into the cathode end of
the stem section. At the boundary of the migration, the donor
particles cause the negatively charged chloroplasts to migrate and
cluster at the cell wall opposite the location of the cathode and
migrating barium ferrite. The grouping here was of an entirely
different spatial patterning then when using donors which enter
the cytoplasm of the cell of the host tissue.
This example serves to teach that in the process of
electrophoretic transduction as described in this invention, the
donor complex can migrate both through the cell free space of the
host tissue, as well as through the plasma membrane into the
cytoplasm of the cell. Furthermore, the nature and ionic strength
of the molecular dipole charges of the donor can significantly
alter the natural, more or less random, spatial distribution of
cell organelles in the cells of tissues being electrophoretically
transduced. Such altered spatial patterns can greatly influence
the probability of the exchange of genetic information between the
cell nucleus and surrounding organelles, and thus provide one
mechanism whereby mutation rates can be significantly increased.
This example also teaches that both organic and inorganic
molecular species can enter the plant cell and interact with the
organelles in a physical and/or biochemical manner. Components
from a macromolecular donor complex produced from plant tissue,
also enter the cell and are active in the organelle spatial
repatterning.
EXAMPLE NINE
Dry seeds of corn (Zea mays) were inserted between the stainless
steel electrodes of the apparatus illustrated in FIG. 8. The
electrodes were covered with filter paper pads moistened with
distilled water. The embroyo end of the seed was placed upward, or
opposite the base plate electrode. At a 45 volt direct current
potential a sharp, well defined uniform line of black pigment was
observed to develop and migrate up the seed if the base plate was
anodic or positive, or down the seed if the base plate was
cathodic or negative.
Since distilled water has a very low ionic content, charge
transport in the seed occurred through the oxidation of the
pigment materials (polyphenols) in the test seed. The migration of
these oxidation products, as testing indicates, is linear with
time. This linear relationship is what would be expected under
conditions of electrophoretic migration. An ionic mobility of
about 0.54.times.10@-6 centimeters squared per volt per second was
observed, a value which is consistent with the rate of movement of
large molecules. Microscopic examination revealed the layer of
oxidation products to extend laterally through the tests into the
outer layers of the endosperm.
This example provides a graphic demonstration of the movement of
large, physiologically related molecules through the plant tissues
under conditions of an electrical potential as applied in the
methods of the present invention.
EXAMPLE TEN
The frequencies of altered enzyme loci producing polymorphism in
corn plants in which Sympolocarpus feotidus is the donor are quite
different from the frequencies when using the soybean root-nodule
extract as the donor. These different allelic responses are
exemplified by commercial electrophoresis tests. In 62 transduced
lines produced in accordance with the method described in
conjunction with FIG. 3, 15 lines were transduced with
Symplocarpus feotidus as the donor, and 47 lines with the soybean
root-nodule as the donor. Table XIV provides a listing of the
number of transduced lines containing a specific enzyme polymorph,
as they occurred within the two donor test groups. Only those
alleles showing positive polymorphism are included in this
listing; those observed to have only a slight variation are
excluded. The enzymes listed are those in which polymorphism
occurred in at least one transduced line.
TABLE XIV
Number of transduced corn linesshowing polymorphism
Soybean
Enzyme S. foetidus root-nodule
ACP (acid phosphatse)
6 11
PGM (phosphogucomutase)
4 1
MDH (malate dehydrogenase)
1 1
PGD (6-phosphogluconate
1 0
dehydrogenase)
PHI (phosphohexose
3 1
isomerase)
GLU (B-glucosidase)
1 0
The data in Table XIV demonstrates that in the soybean root-nodule
lines the majority of the alterations take place at the ACP
alleles. In the lines with S. foetidus as the donor, there were
fewer lines with altered ACP alleles and far more lines involving
other enzymes. The fact that the two enzymes, PGD and GLU revealed
polymorphism in the S. foetidus lines (comprising only 24% of the
total test series) and not in the root-nodule lines (comprising
76% of the test series) again emphasizes the influence of the
donor type on the final genetic response and range of possible
polymorphic alterations that might be achieved by using other
donor types and combinations.
Whatever the mechanism yielding the mutations observed when the
methods of the present invention are employed, the present
invention clearly provides methods for increasing the proportion
of mutants in plant generations. The method of the present
invention are significantly advantageous over the known methods of
recombinant DNA and plasmid fusion techniques, for the reasons
that the precise genetic structure of the chromosomes mutated need
not be elucidated, time and effort need not be wasted in removing
the cell walls, and time and effort need not be wasted in
attempting to grow whole plants from isolated tissues. Instead,
the acceptor plants are whole germinal plants, which after
treatment can be grown in any conventional fashion.
Having described my invention, however, many modifications thereto
will become apparent to those skilled in the area to which it
pertains, without deviation from the spirit of the present
invention, as defined by the scope of the appended claims.
Canadian Journal of Botany, 1970
W.C. Levengood
National Research Council Research Press
Redox currents associated with ion mobility
in stems of plants.
In vivo investigations of bioelectric currents associated with
growth, tissue metabolism, and water responses in the stems of
trees and herbaceous plants are described. This experimentation is
based on a new technique which allows the continuous monitoring of
variations in bioelectric currents related to intertissue redox
conditions. Exploratory studies indicate relationships between
variations in the redox current and turgor conditions in plants. A
correlation between the bioelectric current and the difference in
oxidation potentials between the plant and ground electrodes is
discussed.
In field studies, the current was monitored in different species
of trees over a 1-year interval. Similarities in current
variations occurred in similar species of trees and were examined
in relation to temperature, rainfall, and phenological factors.
The current level, examined in trees as a function of radial
depth, disclosed the maximum output in the cambium layer. In a
study of diurnal variations, a temporal shift in a current maximum
was noted along the tree bole.